Coastal blue carbon: Concept, study method, and the application to ecological restoration
Jianwu TANG1,2*, Shufeng YE3, Xuechu CHEN2, Hualei YANG2, Xiaohong SUN4,Faming WANG1, Quan WEN5 & Shaobo CHEN6 1
Marine Biological Laboratory, Woods Hole 02543, USA; 2 College of Ecology and Environmental Science, East China Normal University; State Key Laboratory of Estuarine and Coastal Research;
Shanghai Key Lab for Urban Ecological Processes and Eco-Restoration, Shanghai 200241, China; 3 East China Sea Branch of State Oceanic Administration, Shanghai 200137, China; 4 Marine College, Shandong University (Weihai), Weihai 264209, China; 5 Environmental Monitoring Center, State Oceanic Administration, Dalian 116203, China; 6 Zhejiang Mariculture Research Institute, Wenzhou 325005, China
Received October 10, 2017; revised January 3, 2018; accepted March 22, 2018; published online April 4, 2018
Abstract
Coastal blue carbon refers to the carbon taken from atmospheric CO?; fixed by advanced plants (including salt marsh, mangrove, and seagrass), phytoplankton, macroalgae, and marine calcifiers via the interaction of plants and microbes; and stored in nearshore sediments and soils; as well as the carbon transported from the coast to the ocean and ocean floor. The carbon sequestration capacity per unit area of coastal blue carbon is far greater than that of the terrestrial carbon pool. The mechanisms and controls of the carbon sink from salt marshes, mangroves, seagrasses, the aquaculture of shellfish and macroalgae, and the microbial carbon pump need to be further studied. The methods to quantify coastal blue carbon include carbon flux measurements, carbon pool measurements, manipulative experiments, and modeling. Restoring, conserving, and enhancing blue carbon will increase carbon sinks and produce carbon credits, which could be traded on the carbon market. The need to tackle climate change and implement China's commitment to cut carbon emissions requires us to improve studies on coastal blue carbon science and policy. The knowledge learned from coastal blue carbon improves the conservation and restoration of salt marshes, mangroves, and seagrasses; enhances the function of the microbial carbon pump; and promotes sustainable aquaculture, such as ocean ranching.
Keywords Coastal, Blue carbon, Ecological restoration, Salt marsh, Mangrove, Seagrass, Microbial carbon pump, Aquaculture carbon sink
Citation: Tang J, Ye S, Chen X, Yang H, Sun X, Wang F, Wen Q, Chen S. 2018. Coastal blue carbon: Concept, study method, and the application to ecological restoration. Science China Earth Sciences, 61: 637-646, https://doi.org/lQ.lQQ7/sll43Q-Q17-9181-x
1. Introduction
The massive emissions of greenhouse gases (GHGs) from human activities, especially CO2 produced by burning fossil fuels over the past century, have led to global climate change, which is one of the most serious challenges facing human beings in the 21st century. Currently, human emissions of CO2 from fossil energy and industrial production release approximately 7.8 PgC yr-1 (IPCC, 2013). These anthropogenic CO2 emissions have undermined the delicate balance between the global ecosystem and the atmosphere and contributed to the increase in atmospheric CO2 from320 ppm to approximately 400 ppm currently over the past 70 years.
Due to the powerful function and the resilience ofthe Earth system, a fraction of the anthropogenic CO2 stays in the atmosphere, and the rest is absorbed by terrestrial and ocean ecosystems, forming the terrestrial and ocean carbon sinks. At present, within the human-induced 7.8 Pg C yr-1 CO2 emissions, approximately 2.3 Pg C yr-1 is absorbed by the oceans and 1.5 Pg C yr-1 is absorbed by land (including 2.6 Pg C yr-1 from net ecosystem absorption and1.1Pg C yr-1 of emissions from land-use change). The remaining of 4.0 Pg C yr-1 stays in the atmosphere (IPCC, 2013). Therefore, increasing the terrestrial and ocean carbon sinks can effectively reduce the atmospheric CO2 concentration and consequently mitigate climate change. Studies of the carbon sink and its associated carbon cycles in the terrestrial and ocean systems have been widely conducted, but research on the land-ocean interface that is affected by the ocean tide has just started.
In the most recent 5 years, international blue carbon research has been rising gradually, partially promoted in blue carbon science and policy through the cooperation between governments and the international organizations, such as Conservation International (CI), the International Union for Conservation of Nature (IUCN), the Intergovernmental Oceanographic Commission (IOC), and the United Nations Educational, Scientific and Cultural Organization (UNESCO). The study of blue carbon policy focuses on the linkage between global carbon trading markets and blue carbon planning, the local policy support for blue carbon project implementation, and the establishment of the local blue carbon trading markets, etc. The scientific research of blue carbon concentrates on the mechanism of blue carbon processes and the quantification of blue carbon science in different coastal ecosystems. Overall, the research on blue carbon policy and science is still in its infancy, while the demand from policy making is at the forefront of blue carbon research. China has actively participated in blue carbon projects and is implementing the “China Blue Carbon Program". However, the science and policy for the coastal blue carbon systems have not been well established, and the definition and evaluation methods of blue carbon have not yet been clarified. Therefore, this paper briefly describes the scientific concept and research methods, as well as its application to the ecological protection and restoration of tide- influenced coastal blue carbon.
2. The concept of costal blue carbon
Ocean blue carbon refers to the absorbed and fixed carbon in the ocean from atmospheric CO2 (Nellemann et al., 2009). Here, the “blue carbon" is analogous to the “green carbon" that is fixed by terrestrial ecosystems. The net absorption (the sum of incoming and outgoing fluxes) of ocean blue carbon from the atmosphere is approximately 2.3 Pg C yr-1, while green carbon absorbs approximately 2.6 Pg C yr-1 (IPCC, 2013). Traditionally, ocean blue carbon is primarily fixed through the physically dissolved carbon pump (atmospheric CO2 is dissolved into seawater), the biological carbon pump (marine phytoplankton absorbs and transforms CO2 through photosynthesis and then deposits it into the seabed), and the marine carbonate pump (the carbon is absorbed, transformed and released by shellfish, coral reefs and other marine organisms) (Mcleod et al., 2011; IPCC, 2013). Terrestrial green carbon is fixed mainly through the terrestrial higher plants that absorb, convert, and fix CO2 via photosynthesis as biomass or into soil.
Coastal blue carbon lies between the ocean blue carbon and terrestrial green carbon. The coastal zone, controlled by seawater and tides, hosts wetland plants (salt marsh and mangrove) and marine plants (seagrass). During recent years, coastal blue carbon has been defined as the carbon fixed by coastal plants, including salt marshes, mangroves, and seagrasses (Mcleod et al., 2011; Zhang et al., 2015; Howard et al., 2017). The underlying reason for this narrow definition is the large productivity and controllability of salt marshes, mangroves and seagrasses. Large productivity refers to the fact that the carbon sequestration capacity of these three coastal ecosystems is approximately 10 times more than that of terrestrial ecosystems (Mcleod et al., 2011). These ecosystems account for only 0.2% of the ocean area, but the carbon deposits in sediments account for approximately 50% of the total carbon deposits in marine sediments (Duarte et al., 2013). Controllability means that human activities can destroy, protect or restore these systems to decrease or increase blue carbon. Therefore, as an effective mechanism to mitigate climate change, we can incorporate coastal blue carbon into carbon credit and carbon trading systems by calculating the difference between the original carbon pool (baseline) and adjusted carbon pools.
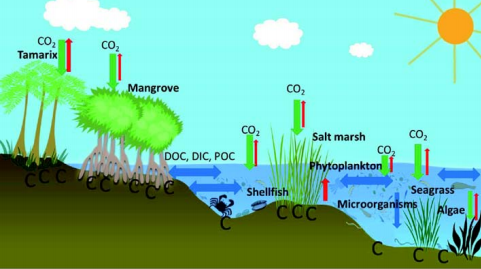
Figure 1 The schematic diagram of coastal blue carbon showing the exchange of CO2 between plants and the atmosphere, seawater and the atmosphere, and the exchange of dissolved organic carbon (DOC), dissolved inorganic carbon (DIC), and particulate organic carbon (POC) within seawater, as well as the accumulation of carbon in sediment. The red arrows indicate the CO2 emissions into the atmosphere, the green arrows indicate the absorption of CO2 into the land/ocean, and the blue arrows indicate the exchange and deposition of DOC, DIC, and POC. Ecosystem types from left to right (from land to sea): tamarisk, mangroves, shellfish, salt marsh, phytoplankton (widely distributed), microorganisms (widely distributed), seagrass, and macroalgae (actually do not necessarily appear in the same region simultaneously).
Here, we attempt to extend the previous definition of coastal blue carbon, which is primarily based on its application of controllability as described above, to a broad sense based on the scientific processes of the carbon cycle in the coastal zone. We contend that coastal blue carbon broadly refers to the carbon that is absorbed from atmospheric CO2 and converted by the higher plants in coastal ecosystems (including salt marshes, mangroves and seagrasses), phytoplankton, algae, and calcifier organisms and then buried in the sediments for the long term under the combined effect of plants and microorganisms. This part of the carbon, as well as the organic carbon that is exported from the coastal zone to offshore areas and the ocean, could be broadly defined as coastal blue carbon. The absorption, conversion and conservation of blue carbon in the coastal zone are complex biological, physical and chemical processes, involving the exchange between land and sea, interaction among plants,animals and microorganisms, and the dynamic space-time transformation between the carbon fluxes and stocks (Figure 1).
Because of their strong photosynthetic capacity and low decomposition rates, salt marshes, mangroves, and seagrasses have high productivity and carbon sequestration capacity per unit area as the major contributors to blue carbon in the coastal zone (Zhang et al., 2015; Zhang et al., 2017). Phytoplankton and algae also absorb CO2 through photosynthesis. Most carbon (mainly POC) immobilized by the biological carbon pump is decomposed by microorganisms, but a small portion is deposited in the sediments of the ocean floor, forming a carbon sink. Meanwhile, a fraction of organic carbon, converted and released by coastal vascular plants, offshore phytoplankton and algae, could be further transformed to recalcitrant dissolved organic carbon (RDOC) and deposited in the ocean for a long time through the process of fixing carbon by the microbial carbon pump (MCP) (Jiao et al., 2008, 2013, 2014; Lechtenfeld et al., 2015; Legendre et al., 2015). Calcified organisms, such as shellfish and coral reefs, both absorb and release CO2 through a complicated process of carbon cycling. The way in which to calculate their carbon fixation capacity as the aquaculture carbon sink is an urgent research field.
The major processes of the blue carbon sink in salt marshes, mangroves and seagrasses, which are the important contributors to coastal blue carbon, could be expressed in the following biochemical reactions:
Photosynthesis:
6CO2+6H2O+light—>C6H2O6+6O2
Respiratory or decomposition:
C6H12O6+6O2—>6CO2+6H2O+heat
The plants can absorb CO2 and light energy from the atmosphere (or water) to produce carbohydrates. Meanwhile, the respiration of plants decomposes carbohydrates and provides the energy for plant growth and reproduction. The dead plants are decomposed into CO2 and other organic and inorganic matter by microorganisms, with the release of biomass and nutrients, and the remaining organic carbon is fixed in the soil, some of which may form recalcitrant carbon stored in soils for the long term (Liang et al., 2017). Although carbon uptake and carbon emissions are always dynamically changing, the system can fix carbon when total photosynthesis is greater than total respiration and decomposition. In the above coastal blue carbon ecosystems, the total photosynthesis is large, and the total decomposition amount is small, and thus, these ecosystems have a great carbon sink capacity. The carbon storage mechanism is mainly attributed to the fact that the anaerobic environment of the sediments inhibits the decomposition process of organic matter, so that a large number of plant residues can be stored for a long time. Recent research has shown that the stable carbon sinks in salt marshes are mainly composed of lignin, which originates from lignin and cellulose tissues in plants. In addition, other recalcitrant carbon in coastal sediments may be due to transformation and sequestration via microbial carbon pumps, similar to the terrestrial ecosystems, where massive labile litter could be converted to stable carbon sinks in soils via the sequestration of soil microbial carbon pumps (Liang et al., 2017).
In general, the blue carbon sequestration capacity per unit area of the higher plants in coastal ecosystems is much larger than that in both terrestrial and ocean ecosystems. Recent reviews have shown that the average carbon sink capacities were 218, 226 and 138 g C m 2 yr 1 in salt marshes, mangroves, and seagrasses, respectively, compared with terrestrial forests of only 5 g C m-2 yr-1 or less (Mcleod et al.2011). According to satellite remote sensing data, the annual average primary productivity of the oceans was approximately 140 g C m-2 yr-1 (Field et al., 1998).
2.1Salt marsh
Salt marshes are ecological buffer zones between the land and ocean, with high productivity, rich biodiversity and extremely important ecosystem services (Chen et al., 2017). Currently, the salt marshes in China are dominated by Phragmites spp., Suaeda glauca, Scirpus mariqueter and Spartina alterniflora, with the total area ranging from 1207 to 3434 km2, storing approximately 550 Pg C and accounting for 15-30% of the total land-based carbon stocks (Guan,2012). Previous studies have shown that the annual carbon sequestration rate of Phragmites australis, Spartina alter- niflora and S. trifoliata on the south bank of Hangzhou Bay were 380%, 376% and 55.5%, respectively, of the average carbon sequestration rates of terrestrial vegetation in China, and 463%, 458% and 67.7% of the average global vegetation carbon sequestration rates, respectively (Shao et al., 2013). Taking the coastal wetland at Chongming Island as an example, the annual carbon sequestration of Phragmites marsh was 1.02 0.12 kg m-2 yr-1, that of S. alterniflora marsh was 1.32 0.10 kg m-2 yr-1, and that of S. mariqueter marsh was 0.33 0.05 kg m-2 yr-1, with total sequestration rates of approximately 0.25 Tg yr-1 on this island (Wang et al., 2014). In the wetlands of the Yellow River Delta, the annual carbon sequestration of P. australis marsh was 0.70 0.16 kg m-2 yr-1, and the annual total carbon sequestration by wetland vegetation in this area reached up to 0.3 Tg yr-1 (Zhang et al., 2012). According to field surveys, the total carbon sequestration in the 0-1.2 m of salt marsh and mangrove soils in the coastal United States is estimated to be 0.87 Pg C (Nahlik and Fennessy, 2016). A coastal salt marsh with an area of 0.25 km2 in North Carolina wetlands absorbed CO2 equivalent to the emissions from 28000 liters of gasoline combustion each year (Davis et al., 2015).
The study of blue carbon in coastal wetlands in China started in the 1990s and mainly focused on the C, N, O, and S cycles, greenhouse gas emissions, and global climate change (Zheng et al., 2013). Globally, studies on blue carbon in salt marshes have mainly focused on the source and sink processes of greenhouse gases, the effect of global change on wetland greenhouse gas emissions (Brannon et al., 2016), the impact of global change on wetland ecosystem structure and functions and other related issues. Field-based real-time monitoring, remote sensing, and mathematical modeling have gradually been applied to studying large-scale wetland carbon.
2.2Mangroves and tamarisk wetlands
Mangroves are a kind of special vegetation composed mainly of Rhizophoraceae species that live in the tidal zone of tropical and sub-tropical coasts. Mangroves are generally distributed in coastal wetlands between 32 S and 32 N. There are approximately 132000 km2 of mangroves worldwide, accounting for 0.4% of the land area on the earth, while the total area of existing mangroves in China is approximately 220 km2, which is mainly distributed along the east and southeast coasts (Lin, 2001). Mangroves, with a strong root system, are the first frontier of coastal shelterbelts and important habitats for seabirds, fish and shrimps, contributing to maintaining coastal ecological balance (Lin, 2001). In recent years, studies on carbon storage and the sequestration of mangrove wetlands have been conducted globally, and some studies further explored the controlling mechanism of carbon cycling in mangrove wetlands to provide scientific recommendations for the protection and utilization of mangrove wetlands. It has been reported that the global carbon sequestration capacity of mangrove wetlands is 0.18 Pg C yr-1, of which the average soil carbon storage in tropical mangrove wetlands up to 3 meters in depth in Southeast Asia was as high as 102.3 kg C m-2 (Donato et al., 2011). The mean carbon sequestration of mangroves in China ranged from 209 to 661 g C m-2 yr-1 (Zhang et al.,2013). In a study of the Jiulong River estuary in China, the net C sequestration of 24-year-old and 48-year-old Kandelia candel mangroves were 1851 and 701 g C m-2 yr-1, respectively, which showed a decreasing trend after the peak growth period, but were still much higher than those of the coniferous and conifer-broadleaf mixed forests (258 g C m-2 yr-1) in subtropical China (Jin et al., 2013).
Tamarisk wetlands are the other important part of the ecological project “South Mangroves and North Tamarisk" in China. Xie et al. (2017) studied the vegetation carbon storage of a tamarisk wetland in Laizhou Bay and found that the vegetation biomass was 940.0 g m-2 and the vegetation carbon storage was 393.1 g m-2. Li et al. (2017) used tamarisk to restore bare coastal wetlands and found that the carbon sequestration of soil and vegetation in the tamarisk ecosystem was 1182 g m-2 after 10 years of a plantation, with an annual C sequestration rate of approximately 100.8 g m-2 yr-1.
2.3Seagrass meadows
large seagrass meadows. It is a habitat for benthos, juvenile larvae and seabirds. There are 10 kinds of seagrass meadows in China, with a total area of87.65 km2, mainly distributed in Shandong, Fujian, Guangdong, Guangxi, Hainan, Taiwan and Hong Kong. Among them, Hainan has the most widely distributed area (Qiu et al., 2014; Li et al., 2010). Seagrass meadows are one of the most effective carbon capture and storage ecosystems on the earth and an important global carbon reservoir. A previous study showed that global seagrass meadows occupy less than 0.2% of the total area of the ocean, but the annual carbon sequestered in seagrass sediments is equivalent to 10% to 15% of total global ocean carbon sequestration. The seagrass organic carbon pool can reach up to 19.9 Pg C, with an annual carbon burial rate of 27.4 Tg C, equivalent to the sum of the carbon sequestration rate in the sediments of mangroves and intertidal salt marshes (Qiu et al., 2014). It was also estimated that the average carbon sequestration rate of seagrass ecosystems in the world was 83 g C m-2 yr-1, approximately 21 times that of tropical rainforests (4 g C m-2 yr-1) (Qiu et al., 2014). In China, research on seagrass blue carbon has gradually started in recent years. In a survey of Sanggou Bay in China, it was found that the net primary production of seagrass plants was approximately 543.5 g C m-2 yr-1. If the carbon contribution from the plants, the cultured clam shellfish and captured particulate carbon from seagrass was pooled together, the total carbon sequestration of the seagrass meadows in Sanggou Bay was 1180 g C m-2 yr-1, totaling 290 Mg C yr-1(Gao et al, 2013).
2.4 Fishery carbon sinks
Studies on carbon sequestration in fisheries contributed by farmed shellfish and macroalgae have been conducted in China for more than a decade (Zhang et al., 2005; Tang and Liu, 2016). The calcification of shellfish and coral reefs affects the ocean's carbon cycle. The calcification process in the ocean is a two-way complex process, mainly in the following reaction:

Here, the shell formation process releases CO2 gas (the rightward reaction), while the shell decomposition process (the leftward reaction) absorbs CO2. However, considering the full carbon cycle, the 2HCO; required for shell formation comes from the dissolved CO2 in seawater, which may in turn come from the dissolution of atmospheric CO2. So, if CaCO3 in shellfish is harvested and stored on land without leaching, shellfish aquaculture would be a potential carbon sink (Ahmed et al., 2017). However, how to measure the HCO3" in the water required for the shell formation and how to quantify the effect of dissolved CO2 in the seawater on the concentration of atmospheric CO2 from the perspective of the calcifier ecosystem is a subject of further study.
Large seaweeds, like planktonic microalgae, absorb sunlight with chlorophyll and fix CO2 through photosynthesis. The fixed organic carbon could be consumed by animals and microorganisms with fast decomposition and little deposition on the ocean floor (Howard et al., 2017). However, macroalgae have a very strong carbon uptake capacity, with a global uptake of approximately 173 TgC yr-1 (Krause-Jensen and Duarte, 2016). If macroalgae are harvested in large quantities and used for food, bioenergy or other purposes, the release of carbon can be prolonged and algae cultivation may be turned into a potential carbon sink. From the perspective of a life-cycle analysis, macroalgae used in human food can replace some agricultural products and reduce the carbon emissions during the production of agricultural products, that is, an integrated analysis of aquaculture-agriculture for the carbon footprint. In addition, 43% of the net primary productivity of macroalgae may be released in the form of DOC and POC in seawater, some of which may be buried in ocean sediments as a carbon sink (Krause-Jensen and Duarte, 2016). With the popularization and application of “marine pastures" in China, it is urgent to intensify studies on the mechanism of carbon sequestration in marine pastures to promote sustainable marine pastures.
2.5 Microbial carbon pump
Phytoplankton is the major contributor to ocean primary productivity, especially in eutrophic coastal ecosystems, where phytoplankton abundance and diversity are much higher than in the open sea. Previous studies have shown that the annual average productivity of global marine phytoplankton accounts for 46.2% of the surface primary productivity on the earth (Field et al., 1998). Marine phytoplankton grows at a higher rate than land plants, up to 105 times higher than terrestrial plants (Marba et al., 2007). In addition, because of the diversity in the structure of coastal ecosystems, primary productivity can be used rapidly by other organisms and then transformed and transferred in the relatively long food chain, slowing down the decomposition of primary productivity to CO2. The reuse and decomposition of phytoplankton in the ocean have produced a large amount ofPOC and DOC.
The biological carbon pump uses the organic carbon sourced from the photosynthesis of phytoplankton, transports and transforms the carbon through the micro-food web and food chain, and ultimately deposits it to the seabed primarily as POC. Therefore, the biological carbon pump is also referred to as the biological deposition pump. Globally, among the carbon captured through photosynthesis, 45% is stored in terrestrial ecosystems as green carbon, and the remaining 55% is stored in the ocean by phytoplankton and coastal blue ecosystems (Nellemann et al., 2009). In recent years, the concept of the biological carbon pump was extended by Nianzhi Jiao and his colleagues by introducing the concept ofthe microbial carbon pump (MCP) and describing the fate of marine organic carbon from the perspective of the microbial and molecular biological mechanism. The microbial carbon pump refers to the complicated process in which the DOC and POC fixed by the biological carbon pump are further transformed into recalcitrant dissolved organic carbon (RDOC) by microorganisms (mainly prokaryotes) and then buried in the ocean for the long term (Jiao et al., 2008, 2011, 2013, 2014; Zhang et al., 2017). Subsequently, international scientists have carried out a large number of mechanistic and quantitative studies to reveal the source and change of the huge ocean carbon stock, especially the generation and evolution of the RDOC stock (Lechtenfeld et al., 2015; Legendre et al., 2015; Osterholz et al., 2015). Recently, the concept of MCP has also been introduced into terrestrial ecosystems to describe the role of microorganisms in converting organic carbon into recalcitrant carbon and retaining it in soils for a long time (Liang et al., 2017).
Because of the huge stock of DOC in the ocean, even if only 5—7% of the DOC is converted to RDOC under the transformation of MCP, the RDOC carbon stock can reach 650 Pg C, similar to the CO2 stock in the atmosphere (Zhang et al., 2017). Legendre et al. (2015) calculated the contribution of global ocean MCP to be 0.2 Pg C yr-1. At present, the quantification of RDOC is mainly through measurements by biological culture experiments or modeling methods.
The role of MCP in carbon sequestration is also applicable to the coastal zone, where human activities can affect the MCP. The transport of nutrients in the coastal zone and ocean acidification caused by climate change can both change the degree of MCP and thus affect the fixation of RDOC (Jiao et al., 2014). The regulation of RDOC in the coastal zone can be linked to carbon credits. Further research should focus on how to improve the functioning of MCP and increase the carbon sink of RDOC in the coastal ocean.
In summary, the broadly defined coastal blue carbon includes different types and processes of carbon fixed by salt marshes, mangroves, seagrasses, fisheries (algae and shellfish aquaculture), and RDOC driven by MCP. The different types of carbon play an important role in maintaining the ecosystem functions and services along the coast. Further studies are needed to reveal the principle processes and regulatory mechanisms of these blue carbons.
3. Quantitative studies on coastal blue carbon
The quantitative methods for coastal blue carbon include monitoring of the carbon budget, manipulative experiments, and modeling research. The monitoring of the carbon budget can be divided into carbon flux observations and carbon stock observations. In Figure 1, the change in the carbon stock in sediment is derived from the changes in the carbon flux (arrows), including vertical and horizontal fluxes as shown in the following equation:

where C is the carbon stock in a system (g C m-2), t is time, and F is all kinds of carbon fluxes (vertical or horizontal carbon sink and source, g C m-2 s-1).
Therefore, the amount of blue carbon can be calculated as the integration of all kinds of carbon fluxes over time or by measuring the carbon stock directly. Monitoring carbon fluxes requires instantaneous observations, which is more complex, with potential large errors, but the advantage is a detailed understanding of the mechanisms and processes of changes in carbon stocks and the creation of a database for carbon modeling. Measurements of carbon stocks are relatively simple and can inform us of the change in carbon stocks for a year or a few years, but they will not inform us of the contributions of seasonal change or of different carbon fluxes to the carbon stock.
The following study methods are mainly used for salt marshes but can also be applied to mangroves and seagrasses (under water), as the measurement principles are similar. The measurement of carbon fluxes and stocks can also be applied to the measurement of fishery carbon stock and DOC or RDOC based on the process of MCP.
3.1Carbon flux measurement
At present, the static chamber method and eddy covariance method are primarily used to measure carbon fluxes.
3.1.1Static chamber method
The principle is to use a certain size of static chamber (transparent and opaque), which is connected to a CO2 or other gas analyzer via a gas pump. The CO2 flux could be measured by the increase in internal CO2 concentration per unit of time after the chamber covers the plant or soil. The basic formula is as follows:

where F is the CO2 flux (卩mol m-2 s-1), Ac is the change in CO2 concentration within a certain period (卩mol m-3), At is the time interval (s), Visthe effective volume ofthe chamber (m3), A is the area covered by the chamber (m2), andH is the effective height of the chamber.
The chamber usually has a small hole to keep the pressure inside the box balanced with that outside (Norman et al., 1992). The errors of the static chamber method have been widely recognized (Davidson et al., 2002; Livingston and Hutchinson, 1995). The chamber errors are induced by the increase or decrease in the CO2 concentration within the chamber, and thus, the changes in the concentration gradient between the inside CO2 and the outside CO2, so that the measured CO2 flux may not be the real CO2 flux if there was no chamber coverage. In addition, the chamber could change the temperature, light and other factors, which will affect the measurement value. Therefore, when using a chamber to measure fluxes, one should select the best time interval (usually within a few minutes) to ensure the inside concentration can be sensed while not changing the environment within the chamber too much.
The net ecosystem production (NEP) can be measured using a transparent chamber, and the total ecosystem respiration (R) can be measured using an opaque chamber, and thus, the total photosynthesis (GPP=NEP+R) can be measured.
At present, the static chamber has two types: manual and automatic (for continuous observation). The instantaneous flux data measured with a chamber should be integrated to the daily sum and annual sum to calculate the annual flux (NER R, and GPP) for a given ecosystem type.
3.1.2Eddy covariance method
The eddy covariance method provides an automated way to conduct flux measurements on a large scale and runs continuously throughout the year to calculate the daily and annual fluxes. This method can only be used in uniform vegetation and flat terrain with a large area (more than 1 ha). It cannot measure manipulative experiments within a small plot. At present, there are hundreds of eddy covariance flux monitoring stations in the world, which are distributed in various kinds of ecosystems and form the global flux network FLUXNET (Baldocchi et al., 2001).
The eddy covariance method is an approximation that is restricted to micrometeorological measurements under specific meteorological and topographic conditions. The basic formula is:
F = WC, (3)
where F (卩mol m-2 s-1) is the average CO2 flux (typically a 30-minute average), w is the vertical wind velocity (m s-1), c is the instantaneous CO2 concentration (卩mol m-3), and w'c is the average of w and c within 30 min. Here, c and w must be measured at a very high frequency (above 10 Hz) to calculate the 30-minute covariance.
3.1.3Lateral flux
To obtain an accurate picture of a system's carbon budget, we have to measure the carbon that is brought in or taken away by tidal and seawater flow, i.e., the lateral flux, including DOC, DIC, and POC. Currently, there is no established method to measure lateral fluxes. Recently, Jianwu Tang and his collaborators conducted lateral flux measurements in the northeastern United States by measuring the seawater flow in the estuaries or tidal creeks, as well as the DOC, DIC, and POC concentrations in the water, and then calculating the lateral fluxes.
3.2Carbon stock measurement
Carbon stocks in salt marsh, mangrove and seagrass ecosystems mainly include the vegetation carbon stocks (including the aboveground and belowground), carbon stocks in soils and sediments, and the biomass in water. The aboveground and belowground vegetation biomass can be calculated using the dry biomass multiplied by the corresponding carbon conversion factor in each part of the plants. Soil carbon stocks and contents can be determined using the carbon analyzer. To measure annual changes in soil or sediment, we can measure the stratification age and carbon content with cored soil samples. The soil age can be quantified by the cesium and lead isotope method (Armentano and Woodwell, 1975). Currently, there is no universal standard for measuring the depth of soil carbon stocks, so the depth of the soil cores should be reported in the statistics.
Carbon stocks in mangrove and seagrass ecosystems are slightly more complex than that in salt marsh ecosystems. Aboveground biomass in mangroves includes magaphaner- ophyte biomass and understory shrub biomass. The biomass of leaves, branches, bark, trunk, flowers and roots in each tree should be calculated according to allometric equations (multiplied by the corresponding carbon content of each component). The total carbon stock value of each component is divided by the quadrat area to obtain the biomass carbon sinks in this quadrat (Peng et al., 2016). In general, mangrove communities have well-developed respiratory roots that needed to be calculated as a single carbon sink. Blue carbon measurements of seagrass should include the seagrass epiphytic biomass. Seagrass epiphytes are organisms that grow on seaweed leaves, including algae, diatoms and other crustaceans. Although epiphytes are typically secondary constituents of organic carbon in seagrass ecosystems, it is still necessary to remove epiphytic organisms from seagrass leaves and analyze them as a separate carbon sink in blue carbon calculations for seagrass (Macreadie et al., 2015).
3.3Manipulative experiment
Field manipulative experiments are conducted to artificially change one or more environmental factors and compare them with the control in order to study the responses of ecosystems to these factors. These experiments can calibrate and validate simulation models. Field manipulation transfers lab experiments and controls over certain parameters directly to the field. Manipulative experiments play an important role in understanding the ecosystem response mechanism, validating models, and predicting future changes. The manipulated factors include temperature, salinity, water level, light, rainfall, CO2 concentration, nitrogen content and others.
The earliest classical manipulative experiments include warming experiments (Melillo et al., 2002, 2011), CO2 addition experiments (Hendrey et al., 1999), nitrogen fertilization experiments (Deegan et al., 2012) and precipitation control experiments (Charles and Dukes, 2009). For example, the long-term (13 years) nitrogen addition experiments on an ecosystem scale in a coastal wetland of New England, USA, showed that nitrogen addition could degrade salt marshes (Deegan et al., 2012), resulting in a reduction of carbon sequestration. A study in the same area showed that both warming and a reduction in precipitation could significantly increase plant biomass in salt marshes (Charles and Dukes, 2009), an advantage for ecosystem carbon sequestration. Compared with the salt marshes, the ecosystem manipulative experiments in mangroves and seagrass were rarely reported, mainly because those ecosystems are more complex in structure than the salt marshes, the cost of these experiments are very high, and the experimental results were not satisfactory.
The future trend of field manipulative experiments in ecosystems is to conduct multifactorial experiments to examine the driving factors and sensitivity of various parameters and to support the development of models with predictive ability. These process-based wetland models should be incorporated into large-scale Earth SystemModels.
3.4 Model research
The goal of long-term observations of ecosystems and experimental studies is to create process-based mathematical models, which can provide insights into the system processes and make predictions for the future under different climate scenarios. Most models use some easily measurable parameters as inputs, such as meteorological factors, vegetation structures, and other data that can be obtained from remote sensing. For example, Morris et al. (2016) developed the Marsh Equilibrium Model (MEM) to predict the carbon sequestration of salt marshes and their response to sea level rise. However, there are rarely any models for simulating carbon sequestration in mangroves and seagrasses. Current models mostly use global meteorological and vegetation data to estimate the total carbon sequestration in mangrove or seagrass ecosystems (Jardine and Siikamaki, 2014). To study wetland carbon sequestration at the regional scale, the current models still lack sufficient predictive ability.
4. Application of coastal blue carbon to wetland restoration
Exploring, maintaining, and promoting coastal blue carbon ecosystems are an ecological solution, which is one of the most efficient and valuable ways to be explored to mitigate climate change in China. In recent years, reclamation has induced a huge loss of coastal wetlands, especially coastal salt marshes, and negatively affected the carbon sequestration ability. Therefore, how to effectively limit reclamation activities, restore the carbon sequestration functions of coastal wetlands, and evaluate their carbon sequestration rates will be significantly important for China's blue carbon research.
One of the important applications of coastal blue carbon research is to evaluate the carbon sequestration value that is achieved through coastal restoration. Once the methodology for blue carbon quantification is approved, we can obtain carbon credits through the restoration of salt marshes, mangrove forests, and seagrasses with the enhancement of carbon sequestration, and thus promote coastal wetland restoration with a market mechanism (Kroeger et al., 2017).
However, until now, most blue carbon studies have been focused on existing coastal wetlands. Wetland restoration and its relevant blue carbon value have rarely been studied. During the National Twelfth Five-Year Plan period, China is promoting large-scale restoration projects called “Blue bay" and “Southern mangrove/Northern Tamarisk." These projects might provide ideal study cases and practices for coastal blue carbon research. For instance, supported by the “National sea-use fees" and “Central islands and sea protection funds," the Fengxian coastal salt marsh restoration project and the Western Jinsan citizen beach consolidation and restoration project were launch at the Northern Hangzhouwan Bay in Shanghai. Facing the erosion of the shoreline and the extinction of wetlands, these projects aimed to restore the salt marsh landscape by controlling water flow, amending sediment, and transplanting plants. Recently, an urban coastal wetland with an area of 23.3 hectares, named the Yingwuzhou Ecological Wetland, was established. If these sites could be used as pilots to explore the blue carbon effects and control mechanisms after salt marsh restoration, it would be helpful to the establishment of a coastal blue carbon assessment system and provide important scientific and technological support for coastal salt marsh restoration activities in China. It will further our understanding of marine ecology towards blue carbon and promote the implementation of salt marsh restoration worldwide (Chen et al., 2016, 2017).
5. Prospects of coastal blue carbon research
In response to the demand to address global climate change and fulfill China's promises on carbon emissions reduction, scientific and policy research on coastal blue carbon should be strengthened, including protecting the integrity of the structure and functions of salt marshes, mangroves, seagrasses and other coastal ecosystems based on blue carbon theories; limiting the predatory, destructive development activities in coastal areas to prevent the rapid loss of blue carbon on a large scale; and making efforts to restore and rebuild the damaged and degraded coastal ecosystems and focusing on their ability to restore blue carbon to benefit carbon sinks.
We should strengthen the research investment on observation methods of blue carbon fluxes and stocks, blue carbon-friendly ecological restoration technology, and a blue carbon assessment and trading system. We should study the carbon sequestration by salt marshes, mangroves, seagrasses, the microbial carbon pump, and fishery systems. We should accelerate efforts to formulate and promote national and international standards of the monitoring, measurement, assessment and other aspects of blue carbon, valuing coastal blue carbon as an important public resource and integrating it into the marine management system. We should establish relevant laws and regulations for the management of coastal blue carbon resources and incorporate blue carbon into the carbon trading market and system to better protect blue carbon and coastal ecosystems.
Acknowledgements We thank two anonymous reviewers for their valuable comments to improve this paper. Writing of this paper is motivated by the “Internationalforum on Coastal Blue Carbon" held on August 25— 27, 2017 in Wenzhou, and “Blue Carbon International Forum" held on November 4, 2017 in Xiamen, where Jianwu Tang made presentations, and benefited from presentations and discussion in these two meetings. This work was supported by the National Natural Science Foundation of China Overseas and Hong Kong-Macao Scholars Collaborative Research Fund (Grant No. 31728003) and the Shanghai University Distinguished Professor (Oriental Scholars) Program (Grant No. JZ2016006).
References
Ahmed N, Bunting S W, Glaser M, Flaherty M S, Diana J S. 2017. Can greening of aquaculture sequester blue carbon? Ambio, 46: 468-477 Armentano T V, Woodwell G M. 1975. Sedimentation rates in a Long Island marsh determined by 210Pb dating. Limnol Oceanogr, 20: 452- 456
Baldocchi D, Falge E, Gu L, Olson R, Hollinger D, Running S, Anthoni P, Bernhofer C, Davis K, Evans R, Fuentes J, Goldstein A, Katul G, Law B, Lee X, Malhi Y, Meyers T, Munger W, Oechel W, Paw K T, Pi- legaard K, Schmid H P, Valentini R, Verma S, Vesala T, Wilson K, Wofsy S. 2001. FLUXNET: A new tool to study the temporal and spatial variability of ecosystem-scale carbon dioxide, water vapor, and energy flux densities. Bull Amer Meteorol Soc, 82: 2415-2434 Brannon E Q, Moseman-Valtierra S M, Rella C W, Martin R M, Chen X,
Tang J. 2016. Evaluation of laser-based spectrometers for greenhouse gas flux measurements in coastal marshes. Limnol Oceanogr Methods, 14: 466-476
Charles H, Dukes J S. 2009. Effects of warming and altered precipitation on plant and nutrient dynamics of a New England salt marsh. Ecol Appl, 19: 1758-1773
Chen X C, Dai W Q, Huang C J, Pan L P, Wu W, You W H. 2017. Design of Compound Ecological Purification System for Preserving Water Quality of Shanghai Yingwuzhou Wetland (in Chinese). China Water Waster Water, 33: 66-70
Chen X C, Gao R F, Tang J W. 2016. Basic views and technological methods of salt marsh restoration and its progress in implementation (in Chinese). Mar Environ Sci, 3: 1-18
Davis J L, Currin C A, O'Brien C, Raffenburg C, Davis A. 2015. Living shorelines: Coastal resilience with a blue carbon benefit. Plos One, 10: e0142595
Davidson E A, Savage K, Verchot L V, Navarro R. 2002. Minimizing artifacts and biases in chamber-based measurements of soil respiration. Agric For Meteorol, 113: 21-37
Deegan L A, Johnson D S, Warren R S, Peterson B J, Fleeger J W, Fa- gherazzi S, Wollheim W M. 2012. Coastal eutrophication as a driver of salt marsh loss. Nature, 490: 388-392
Donato D C, Kauffman J B, Murdiyarso D, Kurnianto S, Stidham M, Kanninen M. 2011. Mangroves among the most carbon-rich forests in the tropics. Nat Geosci, 4: 293-297
Duarte C M, Losada I J, Hendriks I E, Mazarrasa I, Marba N. 2013. The role of coastal plant communities for climate change mitigation and adaptation. Nat Clim Change, 3: 961-968
Field C B, Behrenfeld M J, Randerson J T, Falkowski P. 1998. Primary production of the biosphere: Integrating terrestrial and oceanic components. Science, 281: 237-240
Gao Y P Fang J G, Tang W, Zhang J H, Reng L H, Du MR. 2013. Seagrass meadow carbon sink and amplification of the carbon sink for eelgrass bed in Sanggou Bay (in Chinese). Prog Fish Sci, 1: 17-21
Guan D M. 2012. Coastal Wetland in China (in Chinese). Beijing: Ocean Press
Hendrey G R, Ellsworth D S, Lewin K F, Nagy J N. 1999. A free-air enrichment system for exposing tall forest vegetation to elevated atmospheric CO?. Glob Change Biol, 5: 293-309
Howard J, Sutton-Grier A, Herr D, Kleypas J, Landis E, Mcleod E, Pidgeon E, Simpson S. 2017. Clarifying the role of coastal and marine systems in climate mitigation. Front Ecol Environ, 15: 42-50
IPCC. 2013. Climate Change 2013: The Physical Science Basis. Cambridge: Cambridge University Press
Jardine S L, Siikamaki J V. 2014. A global predictive model of carbon in mangrove soils. Environ Res Lett, 9: 104013
Jiao N Z, Luo T W, Zhang Y, Zhang R, Tang K, Chen F, Zeng Y H, Zhang Y Y, Zhao Y L, Zheng Q, Li Y L. 2011. Microbial Carbon Pump in the Ocean-from Microbial Ecological Process to Carbon Cycle Mechanism (in Chinese). J Xiamen Univ-Nat Sci, 50: 387-401
Jiao N Z, Robinson C, Azam F, Thomas H, Baltar F, Dang H, Hardman- Mountford N J, Johnson M, Kirchman D L, Koch B P, Legendre L, Li C, Liu J, Luo T, Luo Y W, Mitra A, Romanou A, Tang K, Wang X, Zhang C, Zhang R. 2014. Mechanisms of microbial carbon sequestration in the ocean-future research directions. Biogeosciences, 11: 5285- 5306
Jiao N Z, Zhang C L, Chen F, Kan J J, Zhang F. 2008. Frontiers and technological advances in microbial processes and carbon cycling in the ocean. In: Mertens L P, ed. Biological Oceanography Research Trends. New York: Nova Science Publishers Inc. 215-266
Jiao N Z, Zhang C L, Li C, Wang X Y, Dang H Y, Zeng Q L, Zhang R, Zhang Y, Tang K, Zhang Z L, Xu D P. 2013. Controllingmechanisms and climate effects of microbial carbon pump in the ocean (in Chinese). Sci Sin Terr, 43: 1-18
Jin L, Lu C Y, Ye Y, Ye G F. 2013. Carbon Storage and Fixation by Kandelia candel Mangrove in Jiulongjiang Estuary (in Chinese). J Fujian Forest Sci Tech, 4: 7-11
Krause-Jensen D, Duarte C M. 2016. Substantial role of macroalgae in marine carbon sequestration. Nat Geosci, 9: 737-742
Kroeger K D, Crooks S, Moseman-Valtierra S, Tang J. 2017. Restoring tides to reduce methane emissions in impounded wetlands: A new and potent Blue Carbon climate change intervention. Sci Rep, 7: 11914
Lechtenfeld O J, Hertkorn N, Shen Y, Witt M, Benner R. 2015. Marine sequestration of carbon in bacterial metabolites. Nat Commun, 6: 6711
Legendre L, Rivkin R B, Weinbauer M G, Guidi L, Uitz J. 2015. The microbial carbon pump concept: Potential biogeochemical significance in the globally changing ocean. Prog Oceanography, 134: 432X50
Li S, Fan H Q, Qiu G L, Shi Y J. 2010. Review on research of seagrass
http://engine.scichina.com/doi/10.1007/s11430-017-9181-x
beds restoration (in Chinese). Acta Ecol Sin, 30: 2443-2453
Li X G, Guo K, Feng XH, Liu X J. 2017. Carbon storage of soil-vegetation system under different land use patterns in saline coastal regions (in Chinese). Chin J Eco-Agricul,25: 1580-1590
Liang C, Schimel J P, Jastrow J D. 2017. The importance of anabolism in microbial control over soil carbon storage. Nat Microbiol, 2: 17105
Lin P. 2001. A review on the mangrove research (in Chinese). J Xiamen Univ-Nat Sci, 40: 592-603
Livingston G P, Hutchinson G L. 1995. Enclosure-based measurement of trace gas exchange: applications and sources of error. In: Matson P A, Harris R C, eds. Biogenic Trace Gases: Measuring Emissions From Soil and Water. Oxford: Blackwell Science Ltd. 14-51
Macreadie P I, Trevathan-Tackett S M, Skilbeck C G, Sanderman J, Cur- levski N, Jacobsen G, Seymour J R. 2015. Losses and recovery of organic carbon from a seagrass ecosystem following disturbance. Proc R Soc B, 282: 20151537
Marba N, Duarte C M, Agusti S. 2007. Allometric scaling of plant life history. Proceedings of the National Academy of Sciences of the United States of America, 104: 15777-15780
Mcleod E, Chmura G L, Bouillon S, Salm R, Bjork M, Duarte C M, Lovelock C E, Schlesinger W H, Silliman B R. 2011. A blueprint for blue carbon: Toward an improved understanding of the role of vegetated coastal habitats in sequestering CO2. Front Ecol Environ, 9: 552-560
Melillo J M, Butler S, Johnson J, Mohan J, Steudler P, Lux H, Burrows E, Bowles F, Smith R, Scott L, Vario C, Hill T, Burton A, Zhou Y M, Tang J. 2011. Soil warming, carbon-nitrogen interactions, and forest carbon budgets. Proc Natl Acad Sci USA, 108: 9508-9512
Melillo J M, Steudler P A, Aber J D, Newkirk K, Lux H, Bowles F P, Catricala C, Magill A, Ahrens T, Morrisseau S. 2002. Soil warming and carbon-cycle feedbacks to the climate system. Science, 298: 2173-2176 Morris J T, Barber D C, Callaway J C, Chambers R, Hagen S C, Hopkinson C S, Johnson B J, Megonigal P, Neubauer S C, Troxler T, Wigand C. 2016. Contributions of organic and inorganic matter to sediment volume and accretion in tidal wetlands at steady state. Earth's Future, 4: 110- 121
Nahlik A M, Fennessy M S. 2016. Carbon storage in US wetlands. Nat Commun, 7: 13835
Norman J M, Garcia R, Verma S B. 1992. Soil surface CO2 fluxes and the carbon budget of a grassland. J Geophys Res, 97: 18845-18853
Nellemann C, Corcoran E, Duarte C M, Valdes L, De Young C, Fonseca L, Grimsditch G. 2009. Blue Carbon: A Rapid Response Assessment. United Nations Environment Programme
Osterholz H, Niggemann J, Giebel H A, Simon M, Dittmar T. 2015. Inefficient microbial production of refractory dissolved organic matter in the ocean. Nat Commun, 6: 7422
Peng C J, Qian J W, Guo X D, Zhao H W, Hu N X, Yang Q, Chen C P, Chen L Z. 2016. Vegetation carbon stocks and net primary productivity of the mangrove forests in Shenzhen, China (in Chinese). Chin J Appl Ecol, 27: 2059-2065
Qiu G L, Lin X Z, Li Z S, Fan H Q, Zhou H L, Liu G H. 2014. Seagrass ecosystems: Contributions to and mechanisms of carbon sequestration (in Chinese). Chin J Appl Ecol, 25:1825-1832
Shao XX,LiWH, Wu M, Yang W Y, Jiang KY,YeX Q. 2013. Dynamics of carbon, nitrogen and phosphorus storage of three dominant marsh Plants in Hangzhou Bay coastal wetland (in Chinese). Environ Sci, 34: 3451-3457
Tang Q S, Liu H. 2016. Strategy for Carbon Sink and Its Amplifi cation inMarine Fisheries (in Chinese). Eng Sci, 18: 68-73
Wang S Q, Wang H Q, Fang Y, Li K. 2014. Ability of plant carbon fixation in the coastal wetland of Chongming Island (in Chinese). Chin J Ecol, 33: 915-921
Xie L P, Wang M, Wang B D, Shi X Y, Xin M, Wei Q S, He X P, Guo F. 2017. Distribution pattern and influencing factors of vegetation carbon storage of Tamarix chinense in the coastal wetland of Laizhou Bay, China (in Chinese). Chin J Appl Ecol, 28: 1103-1111
Zhang H B, Luo Y M, Liu X H, Fu C C. 2015. Current researches and prospects on the coastal blue carbon (in Chinese). Scientia Sinica Terrae, 45: 1641-1648
Zhang J H, Fang J G, Tang Q S. 2005. The contribution of shellfish and seaweed mariculture in China to the carbon cycle of coastal ecosystem (in Chinese). Adv Earth Sci, 20: 359-365
Zhang L, Guo Z H, Li Z Y. 2013. Carbon storage and carbon sink of mangrove wetland: Research progress (in Chinese). Chin J Appl Ecol, 24: 1153-1159
Zhang X L, Zhang C H, Xu Z J, Hou X J, Cai Q F. 2012. On the relation between carbon storage and reinforced fixation of the coastal wetland vegetation in the Yellow River delta area (in Chinese). J Safety Environ, 12: 145-149
Zhang Y, Zhao M X, Cui Q, Fan W, Qi J G, Chen Y, Zhang Y Y, Gao K S, Fan J F, Wang G Y, Yan C L, Lu H L, Luo Y W, Zhang Z L, Zheng Q, Xiao W, Jiao N Z. 2017. Processes of coastal ecosystem carbon sequestration and approaches for increasing carbon sink. Sci China Earth Sci, 60: 809-820
Zheng Y M, Niu Z G, Gong P, Dai Y J, ShangGuan W. 2013. Preliminary estimation of the organic carbon pool in China's wetlands (in Chinese). Chin Sci Bull, 58: 170-180
(Responsible editor: Nianzhi JIAO)