Carbon sinks/sources in the Yellow and East China Seas—Air-sea interface exchange, dissolution in seawater, and burial in sediments
Jinming SONG1,2,3,4*, Baoxiao QU1,2,3,4?, Xuegang LI1,2,3,4, Huamao YUAN1,2,3,4, Ning LI1,2,3,4 & Liqin DUAN1,2,3,4
1 Key Laboratory of Marine Ecology and Environmental Sciences, Institute of Oceanology, Chinese Academy of Sciences, Qingdao 266071, China;
2 Function Laboratory of Marine Ecology and Environmental Sciences, Qingdao National Laboratory for Marine Science and Technology,
Qingdao 266237, China;
3 University of Chinese Academy of Sciences, Beijing 100049, China;
4 Center for Ocean Mega-Science, Chinese Academy of Sciences, Qingdao 266071, China
Received September 8, 2017; revised March 7, 2018; accepted April 23, 2018; published online July 9, 2018
Abstract
The sinks/sources of carbon in the Yellow Sea (YS) and East China Sea (ECS), which are important continental shelf seas in China, could exert a great influence on coastal ecosystem dynamics and the regional climate change process. The CO2 exchange process across the seawater-air interface, dissolved and particulate carbon in seawater, and carbon burial in sediments were studied to understand the sinks/sources of carbon in the continental shelf seas of China. The YS and the ECS generally have different patterns of seasonal air-sea CO2 exchange. In the YS, regions west of 124 E can absorb CO2 from the atmosphere during spring and winter, and release CO2 to the atmosphere during summer and autumn. The entire YS is considered as a CO2 source throughout the year with respect to the atmosphere, but there are still uncertainties regarding the exact air-sea CO2 exchange flux. Surface temperature and phytoplankton production were the key controlling factors of the air-sea CO2 exchange flux in the offshore region and nearshore region of the YS, respectively. The ECS can absorb CO2 during spring, summer, and winter and release CO2 to the atmosphere during autumn. The annual average exchange rate in the ECS was?4.2 3.2 mmol m?2 d?1 and it served as an obvious sink for atmospheric CO2 with an air-sea exchange flux of 13.7 106 t. The controlling factors of the air-sea CO2 exchange in the ECS varied significantly seasonally. Storage of dissolved inorganic carbon (DIC) and dissolved organic carbon (DOC) in the YS and the ECS were 425 106 t and 1364 106 t, and 28.2 106 t and 54.1 106 t, respectively. Long-term observation showed that the DOC content in the YS had a decreasing trend, indicating that the “practical carbon sink” in the YS was decreasing. The total amount of particulate organic carbon (POC) stored in the YS and ECS was 10.6 106 t, which was comparable to the air-sea CO2 flux in these two continental shelf seas. The amounts of carbon sequestered by phytoplankton in the YS and the ECS were 60.42 106 t and 153.41 106 t, respectively. Artificial breeding of macroalgae could effectively enhance blue carbon sequestration, which could fix 0.36 106–0.45 106 t of carbon annually. Organic carbon (OC) buried in the sediments of the YS was estimated to be 4.75 106 t, and OC of marine origin was 3.03 106 t, accounting for 5.0% of the TOC fixed by phytoplankton primary production. In the ECS, the corresponding depositional flux of OC in the sediment was estimated to be 7.4 106 t yr?1, and the marine-origin OC was 5.5 106 t, accounting for 5.4% of the phytoplankton primary production. Due to the relatively high average depositional flux of OC in the sediment, the YS and ECS have considerable potential to store a vast amount of “blue carbon.”
Keywords Sink/source of carbon, Air-sea CO2 exchange, Dissolved inorganic carbon, Dissolved organic carbon, Particulate organic carbon, Sediment, Yellow Sea, East China Sea
Citation: Song J, Qu B, Li X, Yuan H, Li N, Duan L. 2018. Carbon sinks/sources in the Yellow and East China Seas—Air-sea interface exchange, dissolution in seawater, and burial in sediments. Science China Earth Sciences, 61: 1583–1593, https://doi.org/10.1007/s11430-017-9213-6
1.Introduction
In December 12, 2015, 195 members of the United Nations Framework Convention on Climate Change (UNFCCC) signed the Paris Agreement, which is another important in- ternational agreement to reduce greenhouse gas emissions following the adoption of the Kyoto Protocol on December 11, 1997. The Paris Agreement aims to respond to the global climate change threat by keeping the global temperature increase this century well below 2 C above pre-industrial levels and to pursue efforts to limit the temperature increase even further to 1.5 C. Carbon dioxide (CO2) emitted by anthropogenic activities (e.g., fossil fuel burning, land-use practices) is among most important reasons for global warming and climate change. Therefore, reducing the release of CO2 and enhancing the absorption and storage of CO2 have been considered as key points in slowing global warming and tackling global climate change. The global ocean covers 71% of the area of the earth. Under the influ- ence of complex biogeochemical dynamics, the carbon cy- cling process in the ocean and the air-sea CO2 exchange vary significantly spatially and temporally. Numerous field sur- veys and model results have been conducted in the past few decades have consistently demonstrated that the global oceanis generally a net sink for atmospheric CO2, estimated at 2.2 108 t C yr?1 (Takahashi et al., 2002) and accounting for approximately 48% of the total carbon emitted by anthro-pogenic activities since the industrial period began during the late 18th century (Sabine et al., 2004). However, there is still a “missing sink” of approximately 1.0 108 t C yr?1 in the global carbon budget (Gifford, 1994). Many researchers have argued that this “missing sink” was probably stored in a productive continental sea or marginal sea (Bauer et al., 2013; Thomas et al., 2004; Walsh, 1991).
Although the continental marginal seas only cover an area of 7–10% of the global ocean, they account for 14–30% of the global ocean primary production, 80% of the organic carbon depositional flux, 75–90% of the riverine discharge, and 90% of the fish catch. The continental marginal seas are not only vital regions for the exchange of material and en- ergy among land, ocean, and atmosphere, but also the area most affected by human activities (Vitousek et al., 1997) because approximately 37% of the global population lives near coastal areas. Therefore, continental marginal seas are of great importance to global carbon cycling processes. In early research, the role of continental marginal seas in the global ocean carbon budget was unclear. Continuous studies conducted during the recent 30 years have indicated that the sink/source for carbon in continental marginal seas varies significantly spatially and temporally. Continental marginal seas can be either a large sink or an obvious source of at- mospheric CO2. This CO2 sink/source term is profoundly determined by specific geophysical conditions and biogeo-ochemical processes (Gruber, 2015; Laruelle et al., 2014). In general, a province-based synthesis suggests continental marginal seas at mid-latitudes are the major sink for atmo- sphere CO2 (?3.3 108 t C yr?1), those in low latitudes are basically the source of CO2 (1.1 108 t C yr?1), and those in the high latitudes absorb or release CO2 seasonally (Cai et al., 2006).
The Yellow Sea (YS) and East China Sea (ECS) are on the western side of the Pacific Ocean and are the most important continental marginal seas in China. The carbon sink/source patterns in the YS and the ECS play vital roles in regulating the coastal ecosystems and regional climate of China, and are of wide concern to oceanographic scientists from around the world (e.g., Chou et al., 2009, 2011; Guo et al., 2015; Song, 2010; Song et al., 2008b; Zhai et al., 2015; Zhang et al., 2010). In this article, we elaborate upon the variation in and key controlling factors of the carbon sink/source in the YS and ECS from the aspects of air-sea CO2 exchange, dissolved and particulate carbon in seawaters, and carbon burial in sediments to better understand the carbon cycling process and its related effects on continental shelf seas.
2.Air-sea CO2 exchange process in the YS and ECS
The air-sea CO2 exchange process is the exchange of carbon dioxide occurring in the interface between the liquid phase seawater surface and the gas phase atmosphere. The study of the air-sea CO2 exchange process by Chinese scientists be- gan in the late 1980s. In 1989, the Chinese Mainland initiated the “Key process of ocean flux in the East China Sea Shelf” and Taiwan launched “The Kuroshio edge exchange pro- cesses study” (KEEP) program. These two groundbreaking projects focused on the physical, chemical, biological, and geological processes occurring in the ECS and the Kuroshio Current (KC) and established a solid foundation for the study of carbon cycling in Chinese coastal seas. During recent decades, Chinese oceanographers have made great efforts to explore the spatial-temporal variation in the carbon sink/ source and the relevant controlling factors in Chinese coastal seas, and have improved our understanding of the carbon cycling process particularly in continental marginal seas (Chen et al., 2008; Chou et al., 2009, 2011; Dai et al., 2013; Gao et al., 2008; Li et al., 2015; Qu et al., 2013a, 2014, 2015, 2017; Song, 2010; Song et al., 2008b; Xue et al., 2011, 2012; Zhai and Dai, 2009; Zhai et al., 2015).
2.1Air-sea CO2 exchange process in the YS
The YS is a semi-enclosed marginal sea between the Chinese mainland and the Korean Peninsula (31 40′–39 50′N, 119 10′–126 50′E). A line running northeastward from theChengshan Cape of the Shandong Peninsula to the Chang- shanchuan of the Korean Peninsula divides the YS into the northern Yellow Sea (NYS) and the southern Yellow Sea (SYS). Observations conducted during four cruises covering all seasons from 2006 to 2007 indicated the NYS acted as a net CO2 source with respect to the atmosphere during sum- mer, autumn, winter, and spring at an efflux rate of 3.38, 1.39, 0.24, and 1.88 mmol m?2 d?1, respectively (Xue et al., 2012). Annually, the NYS can release 0.5 106 t C into the atmosphere. Sea surface temperature generally plays a more important role in annual cycle of air-sea CO2 exchange than biological processes in most parts of the study area. Mean- while, the intrusion of the Yellow Sea Warm Current (YSWC) and the seasonal variation in thermoclines also are important controlling factors regulating the CO2 sink/source terms of the NYS. Of special note is that the NYS can ac- tually absorb atmospheric CO2 in some productive regions with an annual average air-sea exchange flux of
2.33mmol m?2 d?1 based on data from the A4HDYD time
series site (Xu et al., 2016). This opposite finding suggests further investigation is needed to obtain exact results of the air-sea CO2 exchange flux in the NYS.
The understanding of the air-sea CO2 exchange processesin the SYS has changed from a “hypothesis” to a “determi- nation”. During the 1990s, Japanese scientists (Tsunogai et al., 1997, 1999) adopted the results obtained from the “PN line” of the nearby ECS into this sea and believed that the SYS was a sink for atmospheric CO2. However, Korean scientists found that the SYS served as a source for atmo- spheric CO2 (11.0 106 t C annually) based on an experi- mental relationship between temperature and Chlorophyll a (Chl a) (Oh et al., 2000). Furthermore, Chinese researchers found that the SYS could absorb atmospheric CO2 only during spring, but released CO2 during summer, autumn, and winter (Table 1). According to seasonal large-scale field surveys, it was estimated that the net air-sea CO2 flux in the
SYS was 7.4 106 t C, most of which occurred during winter when a vertical mixing process was significant (Xue et al., 2012; Zhang et al., 2010). Obviously, there are prominent spatial-temporal variations and complex controlling factors in the air-sea CO2 exchange processes of the SYS (Qu et al., 2014, 2015, 2017). Results from April 2011 and June, July, and October 2012 showed that the nearshore area of the Shandong Peninsula and Jiangsu Shallow (depth <50 m) was pCO2-supersaturated (pCO2=400–600 atm) as a result of intensive water mixing which brought bottom CO2-rich water to the surface. Conversely, the offshore area of the SYS center (depth <50 m) was pCO2-undersaturated (pCO2<390 atm) during April, June, and October, but supersaturated during July. Phytoplankton production sustained by abun- dant nutrient and suitable hydrodynamic conditions was of great importance for this undersaturated pCO2 (Qu et al., 2014). As for the controlling factors for pCO2 variation,temperature played the dominant role during October, whereas non-temperature factors, such as vertical mixing, the Changjiang plume, and biological activity, were con- sidered the primary controlling factors during June and July. Spatially, the control of temperature on pCO2 was pre- dominant in the offshore SYS; the non-temperature factors were predominant in the shallow nearshore area, particularly along the coast of the Shandong Peninsula and the Jiangsu Shallow (Figure 1; Qu et al., 2014).
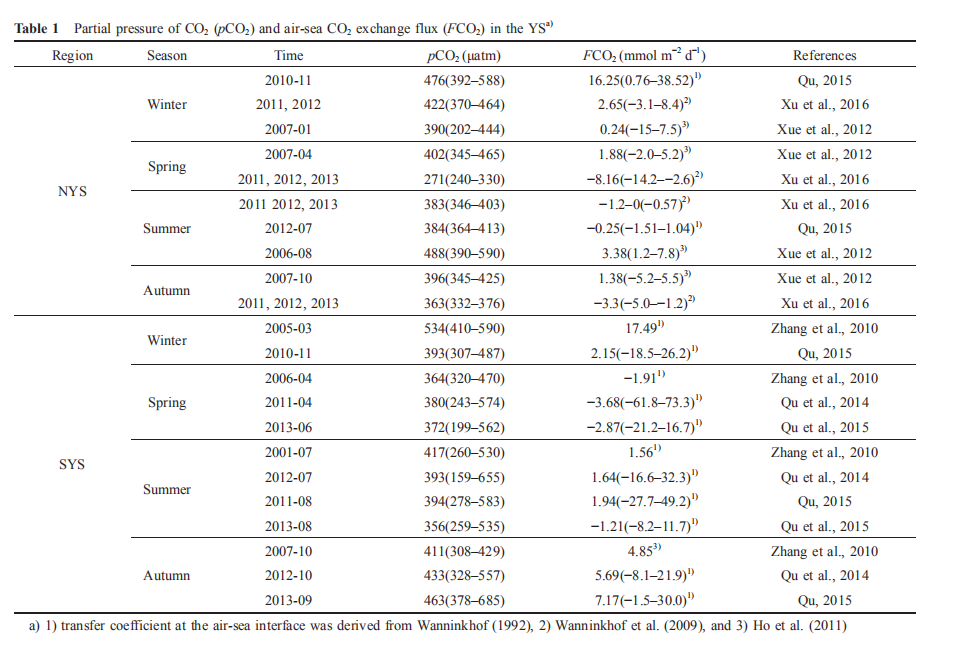
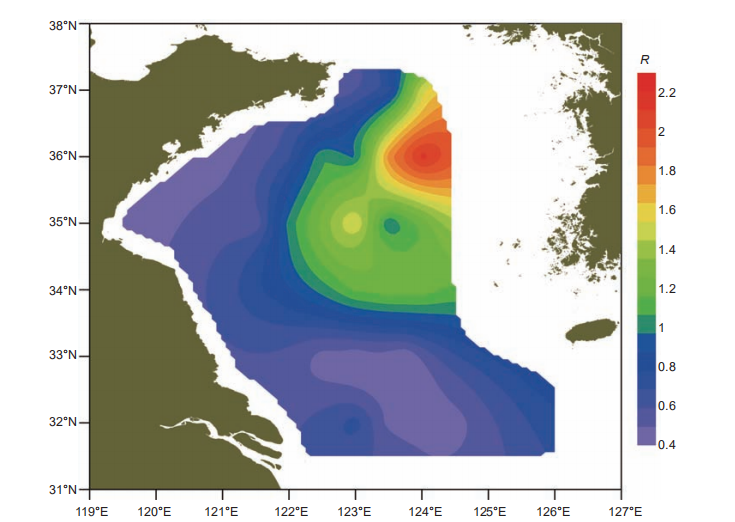
Although considerable research has been implemented during recent decades, some uncertainties remain concerning the CO2 sink/source characteristics of the entire YS (the total of the NYS and the SYS). To better address global climate change, the State Oceanic Administration (SOA) of China has conducted a monitoring project for “Air-sea CO2 Ex- changing Flux” since 2008. This project has also provided abundant observational data to support the study of the car- bon budget of Chinese coastal seas. The latest results of the recent five years have indicated that the YS can absorb CO2 from the atmosphere during winter and spring, while it can release CO2 into the atmosphere during summer and autumn. It seems that the absorption and release of CO2 of the whole YS could achieve a balance (China Marine Environmental Quality Bulletin, 2016).
Summarizing the aforementioned results, it is clear that spatial and seasonal variations in the air-sea CO2 exchange process in the YS are extremely complex. Some uncertainties remain regarding the carbon sink/source patterns of this shelf sea. Hence, long-term, systematic, and comprehensive in- vestigations of the YS air-sea CO2 exchange process are required.
2.2Air-sea CO2 exchange process in the ECS
The ECS (23 00′–33 10′N, 117 11′–131 00′E) is a broadcontinental marginal sea surrounded by the Chinese Main- land, Taiwan, South Korea, and the Kyushu and Ryukyu islands of Japan; 66% of the area is on the continental shelf. During the 1990s, Japanese scientists reported the CO2 sink/ source terms of the ECS based on their limited survey con- ducted along the “PN line” (Tsunogai et al., 1997, 1999). Thereafter, Chinese scientists launched large-scale field surveys to study the air-sea CO2 exchange process in the ECS (Peng et al., 1999; Song et al., 2008b; Wang et al., 2000; Zhang et al., 1997). During the 2000s and 2010s, a series of achievements regarding the CO2 sink/source in the ECS were obtained, including seasonal variation patterns and the con- trolling factors of carbon cycling in the Changjiang estuary and its adjacent region (Chen et al., 2008; Gao et al., 2008; Guo et al., 2015; Li et al., 2015; Song, 2010), differences in seawater carbonate conditions between the ECS and the YS (Qu et al., 2015, 2017), potential evolutionary trends in the ECS under increasing anthropogenic activities and their re-levant influence on the air-sea CO2 exchange flux (Chou et al., 2009; Qu et al., 2013a; Tseng et al., 2011).
Air-sea CO2 exchange processes and their key controlling factors for the ECS vary significantly seasonally and re- gionally. During winter, the nearshore western ECS (west of 125 E), including the Changjiang Estuary and the Hangzhou Bay, is basically a source of CO2 to the atmosphere, while the offshore eastern ECS (east of 125 E) is a sink for atmo- spheric CO2. Intensive vertical mixing induced by the strong winter monsoon and the southward flow of the Yellow Sea Coastal Current (YSCC) are the most important factors for the high pCO2 in the western ECS. At the same time, the eastern ECS, affected by the Taiwan Warm Current (TWC) and KC, has relatively warm seawater temperatures, high phytoplankton production, and stable thermoclines. These factors jointly decrease the pCO2 in this region of the ECS. In particular, Tsunogai et al. (1999) proposed a new term, “continental shelf pump”, as a mechanism for the absorption of CO2 based on their survey conducted in the ECS (27.5 – 31.4 N, 123.0 –128.4 E). This hypothesis suggests that winter cooling along the ECS shelf depresses seawater pCO2 below saturation. Inputs of nutrients and continued CO2 drawdown occur because of primary production, and the subsequent vertical export of organic matter and the hor- izontal export of CO2 as dissolved inorganic carbon (DIC) maintains the CO2 sink status of the continental shelf. This mechanism has been proven along many continental shelves throughout the world.
During spring, the spatial distribution of surface pCO2 in the ECS displays an obvious latitudinal difference, which is delimited by the Changjiang Estuary. In areas north of the Changjiang Estuary, pCO2 still exhibits the same distribution term during winter, namely high pCO2 occurs in the near- shore region and relatively low pCO2 occurs offshore. A line running from 33 N, 126 E to 31 N, 127.6 E divides the ECS into two areas. Water in the coastal western part of the boundary is cold and less saline. Intensive vertical mixing and nearshore upwelling allow this area to release CO2 into the atmosphere (Peng et al., 1999). However, the area off- shore in the southeastern part of the boundary is influenced by the warmer KC. The temperature gradually decreases with the northward flow of seawater and this enhances the solubility of CO2. Therefore, the pCO2 gradually decreases (Zhang et al., 1997). In areas south of the Changjiang Es- tuary, pCO2 shows a new distribution pattern during this season which is opposite to that during winter. The nearshore seawater of this area begins to show low pCO2 values (pCO2<300 atm) and pCO2 values in the offshore seawater remain at 340 atm. The development of the Changjiang Diluted Water (CDW) is probably the cause of this dis- crepancy between the nearshore and offshore seawater.
A significant spatial discrepancy in the CO2 sink/source isalso observed during summer. In general, the nearshore re- gions, such as the Changjiang Estuary, Hangzhou Bay, and the coastal of Fujian-Zhejiang, have relatively high pCO2 and serve as sources of atmospheric CO2. This is probably related to importation of terrestrial discharge. A great amount of terrestrial discharge can not only limit phytoplankton pro- duction by high turbidity but also release dissolved inorganic carbon into the water column via mineralization. Further- more, the middle and eastern shelf of the ECS have a rela- tively low pCO2 and serve as sinks of atmospheric CO2, centered on regions such as 32 N, 123 E, 32 N, 124 E, and 29.67 N, 124 E. The discharge of the CDW, which reaches its peak during summer, is the vital geophysical dynamic for absorption of CO2 in the ECS during summer because it carries abundant nutrients (e.g., nitrate, phosphate, and sili- cate) into the ECS and fuels the photosynthesis of the marine phytoplankton. Consequently, the pCO2 of the surface sea- water decreases due to the primary production (Zhai et al., 2007; Zhai and Dai, 2009). In addition, because it is influ- enced by the KC, the Tsushima Current, and the TWC, the outer shelf of the ECS is generally a CO2 source for the atmosphere with a pCO2 higher than 380 atm (Chou et al., 2009). Relatively high seawater temperature is the demon- strative cause of the release of CO2 (>27 C year round).
The ECS behaves as a net source of CO2 to the atmosphereduring autumn. Specifically, the nearshore region of the ECS can intensively release CO2 into the atmosphere. Influenced by the intense north wind during this season, the CDW and Min-Zhe Coastal Current flow toward the south resulting in significant upwelling. The CO2-enriched bottom seawater is brought to the top layer under the action of upwelling and elevates the concentration of pCO2 in the surface water. In addition, temperature is another important reason for the high pCO2 during this period. During autumn, the northward flowing TWC is quite notable. It brings warm and saline seawater into the ECS and naturally elevates the temperature of the surrounding seawater. Under the function of thermo- dynamic equilibrium, the pCO2 value can increase in a warm environment.
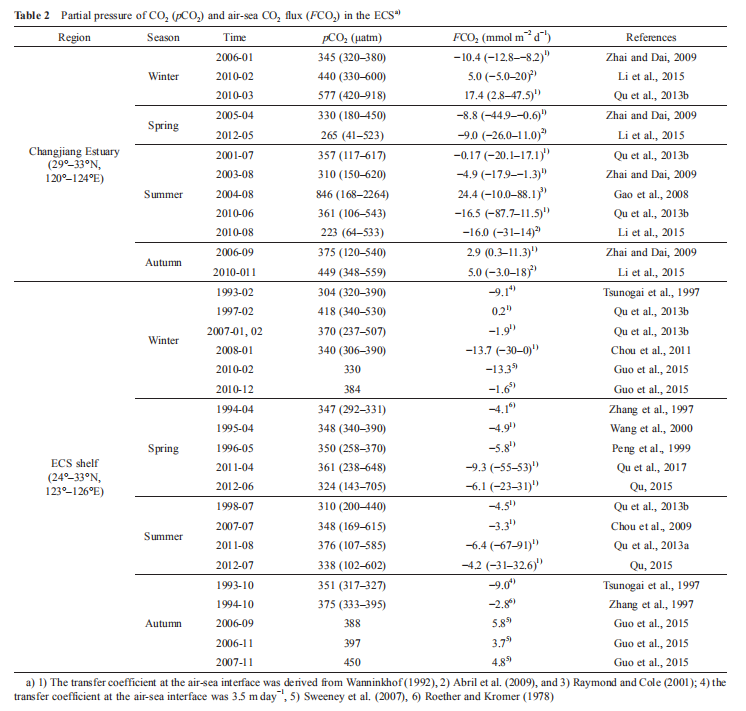
Because of the complexity of the air-sea CO2 exchange process and the limitations of field observation, it is usually quite difficult to evaluate the exact magnitude of the air-sea CO2 flux in a continental marginal sea. Nevertheless, based on the abundant data of recent decades, the ECS is estimated as a net sink for atmospheric CO2 (Table 2). In detail, the ECS can absorb CO2 during winter, spring, and summer, and release CO2 into the atmosphere during autumn, with air-sea exchange flux of ?6.6 6.2, ?6.0 2.0, ?4.6 1.3, and 0.5 6.3 mmol m?2 d?1, respectively. On the whole, with an average air-sea CO2 exchange flux of ?4.2 3.2 mmol m?2 d?1 and the area of continental shelf comprising 0.75 106 km, the ECS can absorb 13.7 106 t C yr?1 annually.
3.Dissolved carbon and particulate carbon in the YS and ECS
Carbon dissolves in seawater usually in the form of DIC, dissolved organic carbon (DOC), and particulate organic carbon (POC) (Song, 2010; Song et al., 2008b). The DIC content of seawater is defined as follows:DIC = CO* + [HCO ] + CO2 , where CO* represents the concentration of all unionized CO2 present as H2CO3 or [CO2]. The amount of inorganic carbon in the ocean accountsfor 60 times that of the atmosphere. During the past10000 years, the ocean has always been the greatest storagefor inorganic carbon (3.8 1019 g) (Falkowski et al., 2000). Furthermore, the DOC in seawater has a potentially great impact on the carbon cycle in the marine system because DOC is a major carbon reservoir (7.5 1017 g), which ac- counts for 17% of the global organic carbon storage (Hedges, 1992). This is comparable to the annual primary productivity of the whole ocean (approximately 5.0 1017 g). A compre- hensive understanding of the cycling of DIC and DOC in the marginal seas is of great significance to the carbon sink/ source of the global ocean. Table 3 summarizes the dissolved carbon in the YS and ECS.

Coastal regions of the YS and ECS usually have a high content of DIC, whereas the DIC concentration in the outershelf of the ECS is relatively low (Chou et al., 2011). This distribution pattern indicates the profound effects of water mass and current systems on regional variations of DIC and the carbon sink/source. Not only that, the Huangpu River usually discharges abundant DIC into the Changjiang Es- tuary, and this is the key reason that the inner estuary ofChangjiang can significantly release CO2 into the atmo- sphere (15.5–34.2 mol m?2 yr?1, Zhai et al., 2007). It is esti- mated that the total storage of DIC in the YS and ECS is 425 106 and 1364 106 t C, respectively (Table 3). Such a large carbon pool has huge potential to increase its carbonsink via regulation.
DOC takes on various forms in the seawater environment,30% of which is carbohydrate, and 12% of which is com- posed of amino acids, uronic acids, aldehydes, and ketone. Abundant research indicates the seasonal variation and re- gional discrepancy in DOC in the YS and the ECS are fairly significant. In general, the average content of DOC during autumn, summer, spring, and winter is 1.99, 1.66, 1.56, and1.53 mg L?1, respectively (Figure 2). The ratio between DOC and POC in the YS is approximately 5:1, implying the dominant role of DOC in the oceanic TOC. Long-term (1997–2015) observations conducted in the SYS indicate there is a reducing trend for DOC content during recent years (Figure 3). As for the ECS, the variation range of DOC in the western part is 0.78–0.90 mg L?1 (Ogawa et al., 2003), whereas the DOC content in the southern ECS is largely greater than 1.02 (Hung et al., 2003). The storages of DOC in the water columns of the YS and ECS are estimated to be 28.2 106 and 54.1 106 t C (Table 3). These storages aregreater than the magnitude of the air-sea CO2 exchange flux but less than the reserves of DIC. The latest research re- garding DOC in the ocean suggests that most DOC could be regarded as recalcitrant dissolved organic carbon (RDOC), which is difficult for phytoplankton to use and has a re- sidence time of from 10–1000 years.
The POC in a marine system includes biomass forms, such as colloidal particles, plankton, and fish, and inanimate ob- jects, such as feces, carapace, and ecdysis (Song et al., 2008b). The POC contents in the YS and the ECS are high during spring (230.0 g L?1), followed by those during summer (147.2 g L?1) and winter (125.9 g L?1), and lowest during autumn (97.4 g L?1) (Figure 4). Because of relatively shallow water depth, the vertical profiles of POC in the YS are quite uniform because of vertical mixing. However, the POC distributions in the Changjiang Estuary show an ob- vious discrepancy between the upper layer and the bottomlayer. This is likely because of the influence of suspended sediment discharge from the Changjiang River. As for the ECS shelf, the combined action of phytoplankton activity and hydrological dynamics jointly control the variation in POC. It is estimated that the total reserves of POC in the YS and ECS are bot approximately 10.6 106 t C (Table 3), which is the same level of the air-sea CO2 exchange flux.
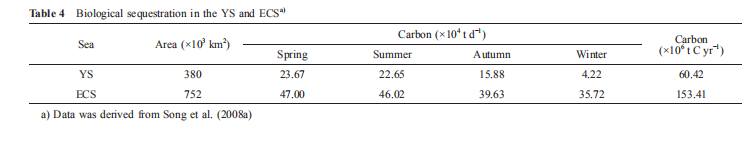
Primary production of marine phytoplankton is a vital process that transforms dissolved carbon into the particulate carbon. The primary production of marine phytoplankton is also known as the biological sequestration. The evaluation of biological sequestration in Chinese marginal seas indicates that the amount of carbon fixed by phytoplankton in the YS is approximately 60.42 106 t C yr?1 and that in the ECS is approximately 153.41 106 t C yr?1 (Tables 3 and 4). Sig- nificant seasonal variations in biological sequestration in the YS and ECS have been observed. Most of the carbon is fixed during spring and summer (Song et al., 2008a). Fishing and mariculture can efficiently enhance the carbon sink strength. During recent years, the yield of large-size economic sea- weeds in China marginal seas has been approximately 1.20 106–1.50 106 t C, thus the fixed carbon is approxi- mately 4.0 105 t C. If the seaweed yield amount increases by 5% every year in the Chinese marginal seas, the fixed carbon amount of the large-sized economic seaweeds may reach 9.3 105 t C in 2020. Therefore, algal breeding is an im- portant technique to increase the marine carbon sink with multiple values (Song, 2011).
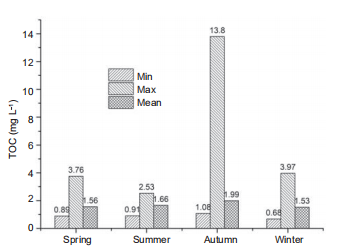
Figure 2 Seasonal average contents of TOC in the YS.
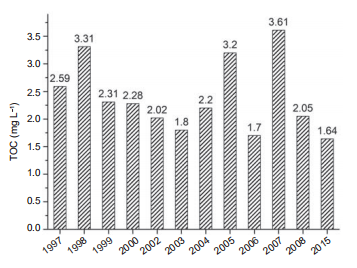
Figure 3 Variation in TOC in the SYS from 1997 to 2015.
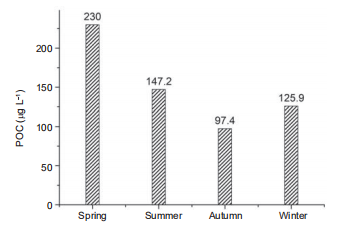
Figure 4 Seasonal average contents of POC in the YS and ECS.
4.Carbon burial in the sediments of the YS and ECS
Atmospheric CO2 enters the ocean via the air-sea interface. It dissolves in seawater, reacts with other constituents, and is utilized by marine organisms. Finally, a part of the particu- late carbon settles and is buried in the sediment, dropping out of the marine carbon cycle for quite a long time. Burial in sediment is considered as the final pool of atmospheric CO2 (Burdige, 2005). Although coastal and marginal seas cover only 7% of the world’s oceanic area (2.5 107 km2), CO2 uptake in them plays a significant role in the global carbon budget. Because a huge inventory of carbon and rapid se-diment accumulation is observed in these shelves, Hedges and Keil (1995) suggested that approximately 80% of the organic carbon in the marine environment is stored in delta and continental shelf sediments (approximately 1.30 1014 g C yr?1), which accounts for one-fifth of the total carbon absorbed by the global ocean (Thomas et al., 2004). Consequently, these shelf seas play a key role in the global carbon cycle by linking terrestrial, oceanic, and atmospheric carbon reservoirs. Terrestrial material input, hydrological conditions, primary production, and sedimentary rate are all vital controlling factors for carbon burial in marine sedi- ments.
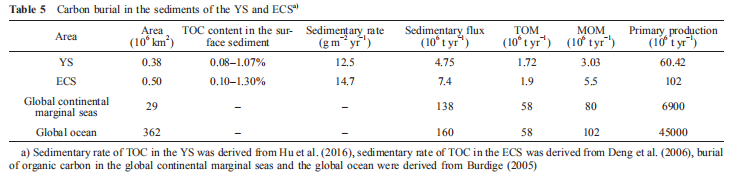
The YS and ECS comprise an important continental mar- ginal portion of the western Pacific Ocean, not only because it links the world’s largest continent (Asia-Europe) and ocean (Pacific Ocean), but also because of the high primary pro- duction and huge riverine sediment discharge in this region. Generally, organic carbon content in sediment is the most important index for carbon burial in the ocean. On average, the concentration of organic carbon in surface sediments of the YS and ECS range from 0.08–1.07% and 0.10–1.30%, respectively (Deng et al., 2006; Hu et al., 2016). Impacted by the geochemical conditions of sediment and the hydrological dynamics of the water column, organic carbon in a mud area of the middle YS, the Changjiang Estuary, and the coastal region of Min-Zhe is generally higher than that in sandy areas. Furthermore, sediment rate is the critical dynamic condition that controls the depositional flux of organic car- bon. According to historical research data, the YS and ECS have a similar sedimentation rate, 12.5 and 14.7 g C m?2 yr?1, respectively (Deng et al., 2006; Hu et al., 2016) that is higher than the average level of the global ocean (approximately 4 g C m?2 yr?1, Berner, 1982). Consequently, the depositional flux of organic carbon in the YS and ECS is calculated as 4.75 106 t and 7.40 106 t C yr?1, respectively, approximately accounting for 9% of the total depositional flux of organic carbon in the global ocean (Burdige, 2005; Table 5). Con- sidering the area of the YS and ECS covers only approxi- mately 3% of the world continental marginal area (2.5 107 km2), the burial of carbon in the YS and ECS is fairly significant.
Organic carbon originating from different sources is usually provided in various proportions in marine sediment. In general, the organic carbon in sediment can be identified as terrestrial organic matter (TOM) and marine organic carbon (MOM) according to the different origins. TOM in- cludes vegetal organic matter in modern time and aged soil organic carbon, while MOM is produced by primary pro- duction of marine phytoplankton. A summary of the data of recent research shows there is 1.72 106 t C yr?1 of TOM and 3.03 106 t C yr?1 of MOM depositing in the YS, whereas the TOM and MOM deposited in the ECS is 1.9 106 and 5.5 106 t C yr?1, respectively (Table 5). The distribution of TOM in the YS and ECS are controlled profoundly by riv- erine discharge and hydrological dynamics. The Changjiang River and the Yellow River are the most important sources of river discharge for the Chinese coastal seas, which discharge 1.0 109 and 4.8 108 t yr?1 of suspended sediment into the YS and the ECS, respectively (Milliman and Meade, 1983). Therefore, coastal seas near the Yellow River estuary, the Changjiang Estuary, and the Subei Shallow have high con- tents of TOM in their surface sediment. With increasing offshore distance, the inputting of TOM is reduced gradually, and the percentage of TOM in the sediment decreases si- multaneously. As for MOM in sediment, high content is usually found in the middle YS, the Changjiang Estuary and its adjacent area, and the Min-Zhe coastal region. These re- gions are normally provided a high level of phytoplankton primary production and serve as the main body for the burial of carbon in the YS and the ECS. A comparison study in- dicates the proportions of MOM in the sediment could ac- count for 5.0% and 5.4% of the phytoplankton primary production, which are obviously higher than the average value of the global ocean (Table 5). In particular, research conducted on sedimentary organic matter in coastal areas of the ECS indicates anthropogenic activities have played a critical role in the carbon burial of continental marginal seas during the past 100 years (Cao et al., 2017). The increase in nutrient supply caused by more intensive human activities (e. g., fertilization and sewage discharge), along with a strengthened KC and East Asian Winter Monsoon (EAWM), have led to an enhanced MOM contribution and increased sedimentary organic matter abundance in the ECS sediments over the past 100 years. In contrast, a significant reduction in TOM contribution is attributed to intensive dam construction following the 1960s. The aforementioned finding not only showed that large-scale human activities have affected thecarbon burial in continental shelf sediment, but also provided new insights for improving carbon storage in shelf seas.
5.Conclusion and prospects
Since the beginning of the 21st century, the contradiction between rapid development of human society and the limited earth environment has become gradually significant. The subsequent consequences of global change, such as global warming, melting glaciers, ocean acidification, sea-level rise, frequent extreme weather, thermohaline circulation weakening, and species extinction have threated the ecolo- gical safety of the natural environment and sustainable de- velopment of human society. Consequently, research regarding global change has become an important focus for scientists throughout the world. Among various projects re- garding global change, the biogeochemical cycling of carbon has always been the vital focus. Understanding the role of carbon in the global cycling of material and energy, and the related key controlling mechanisms, could show the inter- relationship between human activities and the natural en- vironment and help to establish an efficient solution program to address climate change.
The carbon sink/source of continental marginal seas is generally influenced by various processes, such as anthro- pogenic activities, hydrological dynamics, terrestrial riverine discharge, and phytoplankton growth. To obtain an exact estimation of carbon budget in continental marginal seas and understand the important roles of continental marginal seas in global carbon cycling processes, intensive cooperation of diverse subjects, appropriate arrangement of field survey, and stable economic support are of great importance. Fur- thermore, a comprehensive understanding of carbon sink/ source characteristics in different marginal seas requires long-term observation and continuous survey. In the future, we need to restructure the structure and policy of the marine economy, research methods of biological carbon sequestra- tion and establish detailed regulations of “carbon trading”, for the sake of “reducing carbon release” and “increasing carbon sinking”.
Acknowledgements We appreciate the two anonymous reviewers for their valuable comments. This work was supported by the Strategic Priority Research Program of the Chinese Academy of Sciences (Grant No. XDA11020102), the Joint Fund between the National Natural Science Foundation of China and Shandong Province (Grant No. U1606404), and the Program for Aoshan Excellent Scholars of Qingdao National Labora- tory for Marine Science and Technology (Grant No. 013ASTP-OS13).
References
Abril G, Commarieu M V, Sottolichio A, Bretel P, Gu rin F. 2009. Tur- bidity limits gas exchange in a large macrotidal estuary. Estuar Coast Shelf Sci, 83: 342–348
Bauer J E, Cai W J, Raymond P A, Bianchi T S, Hopkinson C S, Regnier P A G. 2013. The changing carbon cycle of the coastal ocean. Nature, 504: 61–70
Berner R A. 1982. Burial of organic carbon and pyrite sulfur in the modern ocean; its geochemical and environmental significance. Am J Sci, 282: 451–473
Burdige D J. 2005. Burial of terrestrial organic matter in marine sediments: A re-assessment. Glob Biogeochem Cycle, 19: GB4011
Cai W J, Dai M, Wang Y. 2006. Air-sea exchange of carbon dioxide in ocean margins: A province-based synthesis. Geophys Res Lett, 33: L12603
Cao Y Y, Xing L, Zhang T, Liao W H. 2017. Multi-proxy evidence for decreased terrestrial contribution to sedimentary organic matter in coastal areas of the East China Sea during the past 100 years. Sci Total Environ, 599-600: 1895–1902
Chen C T A, Zhai W D, Dai M H. 2008. Riverine input and air-sea CO2 exchanges near the Changjiang (Yangtze River) Estuary: Status quo and implication on possible future changes in metabolic status. Cont Shelf Res, 28: 1476–1482
Chou W C, Gong G C, Sheu D D, Hung C C, Tseng T F. 2009. Surface distributions of carbon chemistry parameters in the East China Sea in summer 2007. J Geophys Res, 114: C07026
Chou W C, Gong G C, Tseng C M, Sheu D D, Hung C C, Chang L P, Wang L W. 2011. The carbonate system in the East China Sea in winter. Mar Chem, 123: 44–55
Dai M H, Cao Z M, Guo X H, Zhai W D, Liu Z Y, Yin Z Q, Xu Y P, Gan J P, Hu J Y, Du C J. 2013. Why are some marginal seas sources of atmospheric CO2? Geophys Res Lett, 40: 2154–2158
Deng B, Zhang J, Wu Y. 2006. Recent sediment accumulation and carbon burial in the East China Sea. Glob Biogeochem Cycle, 20: GB3014 Falkowski P, Scholes R J, Boyle E, Canadell J, Canfield D, Elser J, Gruber
N, Hibbard K, Hogberg P, Linder S, Mackenzie F T, Moore B, Pedersen T, Rosenthal Y, Seitzinger S, Smetacek V, Steffen W. 2000. The global carbon cycle: A test of our knowledge of earth as a system. Science, 290: 291–296
Gao X L, Song J M, Li X G, Li N, Yuan H M. 2008. pCO2 and carbon fluxes across sea-air interface in the Changjiang Estuary and Hangzhou Bay. Chin J Ocean Limnol, 26: 289–295
Gifford R. 1994. The global carbon cycle: A viewpoint on the missing sink.
Funct Plant Biolo, 21: 1–15
Gruber N. 2015. Carbon at the coastal interface. Nature, 517: 148–149 Guo X H, Zhai W D, Dai M H, Zhang C, Bai Y, Xu Y, Li Q, Wang G Z.
2015. Air-sea CO2 fluxes in the East China Sea based on multiple-year underway observations. Biogeosci Discuss, 12: 5123–5167
Hedges J I, Keil R G. 1995. Sedimentary organic matter preservation: An assessment and speculative synthesis. Mar Chem, 49: 81–115
Hedges J I. 1992. Global biogeochemical cycles: Progress and problems.
Mar Chem, 39: 67–93
Ho D T, Wanninkhof R, Schlosser P, Ullman D S, Hebert D, Sullivan K F. 2011. Toward a universal relationship between wind speed and gas exchange: Gas transfer velocities measured with 3He/SF6 during the Southern Ocean Gas Exchange Experiment. J Geophys Res, 116: C00F04
Hu L M, Shi X F, Bai Y Z, Qiao S Q, Li L, Yu Y G, Yang G, Ma D Y, Guo
Z G. 2016. Recent organic carbon sequestration in the shelf sediments of the Bohai Sea and Yellow Sea, China. J Mar Syst, 155: 50–58
Hung J J, Chen C H, Gong G C, Sheu D D, Shiah F K. 2003. Distributions, stoichiometric patterns and cross-shelf exports of dissolved organic matter in the East China Sea. Deep-Sea Res Part II-Top Stud Oceanogr, 50: 1127–1145
Laruelle G G, Lauerwald R, Pfeil B, Regnier P. 2014. Regionalized global budget of the CO2 exchange at the air-water interface in continental shelf seas. Glob Biogeochem Cycle, 28: 1199–1214
Li X G, Song J M, Yuan H M, Li N, Duan L Q, Qu B X. 2015. CO2 flux and seasonal variability in the turbidity maximum zone and surrounding area in the Changjiang River estuary. Chin J Ocean Limnol, 33: 222– 232
Milliman J D, Meade R H. 1983. World-wide delivery of river sediment to the oceans. J Geol, 91: 1–21
Ogawa H, Usui T, Koike I. 2003. Distribution of dissolved organic carbon in the East China Sea. Deep-Sea Res Part II-Top Stud Oceanogr, 50: 353–366
Oh D C, Park M K, Kim K R. 2000. CO2 exchange at air-sea interface in the Huanghai Sea. Acta Ocean Sin, 19: 79–89
Peng T H, Hung J J, Wanninkhof R, Millero F J. 1999. Carbon budget in the East China Sea in spring. Tellus B-Chem Phys Meteorol, 51: 531– 540
Qu B X. 2015. Carbon chemistry parameters and air-sea CO2 flux in the Yellow Sea and the East China Sea: Variations and controls (in Chinese with English abstract). Doctoral Dissertation. Qingdao: Institute of Oceanology, Chinese Academy of Sciences. 120
Qu B X, Song J M, Li X G, Yuan H M, Li N, Ma Q X. 2013a. pCO2
distribution and CO2 flux on the inner continental shelf of the East China Sea during summer 2011. Chin J Ocean Limnol, 31: 1088–1097 Qu B X, Song J M, Yuan H M, Li X G, Li N, Duan L Q, Ma Q X, Chen X. 2013b. Advances of seasonal variations and controlling factors of the
air-sea CO2 flux in the East China Sea (in Chinese with English ab- stract). Adv Earth Sci, 28: 783–793
Qu B X, Song J M, Yuan H M, Li X G, Li N, Duan L Q, Chen X, Lu X. 2015. Summer carbonate chemistry dynamics in the Southern Yellow Sea and the East China Sea: Regional variations and controls. Cont Shelf Res, 111: 250–261
Qu B X, Song J M, Yuan H M, Li X G, Li N, Duan L Q. 2017. Comparison of carbonate parameters and air-sea CO2 flux in the southern Yellow Sea and East China Sea during spring and summer of 2011. J Oceanogr, 73: 365–382
Qu B X, Song J M, Yuan H M, Li X G, Li N. 2014. Air-sea CO2 exchange process in the southern Yellow Sea in April of 2011, and June, July, October of 2012. Cont Shelf Res, 80: 8–19
Raymond P A, Cole J J. 2001. Gas exchange in rivers and estuaries: Choosing a gas transfer velocity. Estuaries, 24: 312–317
Roether W, Kromer B. 1978. Field determination of air-sea gas exchange by continuous measurement of radon-222. Pure Appl Geophys, 116: 476–485
Sabine C L, Feely R A, Gruber N, Key R M, Lee K, Bullister J L, Wan- ninkhof R, Wong C S, Wallace D W R, Tilbrook B, Millero F J, Peng T H, Kozyr A, Ono T, Rios A F. 2004. The oceanic sink for anthropogenic CO2. Science, 305: 367–371
Song J M. 2010. Biogeochemical Processes of Biogenic Elements in China Marginal Seas. In: Lin H F, Zhai Z Y, eds. Hangzhou: Zhejiang Uni- versity Press. 662
Song J M. 2011. Carbon cycling process and carbon fixed by organisms in China marginal seas (in Chinese with English abstract). J Fish Sci China, 18: 703–711
Song J M, Li X G, Yuan H M, Zheng G X, Yang Y F. 2008a. Carbon fixed by phytoplankton and cultured algae in China coastal seas (in Chinese with English abstract). Acta Ecol Sin, 28: 551–558
Song J M, Xu Y F, Hu W P, Ni L Y. 2008b. Biogeochemistry of Carbon in China Seas and Lakes (in Chinese with English abstract). Beijing: Science Press. 533
Sate Oceanic Administration, People’s Republic of China. 2016. China
Marine Environmental Quality Bulletin, 6: 59–60
Sweeney C, Gloor E, Jacobson A R, Key R M, McKinley G, Sarmiento J L, Wanninkhof R. 2007. Constraining global air-sea gas exchange for CO2 with recent bomb 14C measurements. Glob Biogeochem Cycle, 21: GB2015
Takahashi T, Sutherland S C, Sweeney C, Poisson A, Metzl N, Tilbrook B, Bates N, Wanninkhof R, Feely R A, Sabine C, Olafsson J, Nojiri Y. 2002. Global sea-air CO2 flux based on climatological surface ocean pCO2, and seasonal biological and temperature effects. Deep-Sea Res Part II-Top Stud Oceanogr, 49: 1601–1622
Thomas H, Bozec Y, Elkalay K, de Baar H J W. 2004. Enhanced open ocean storage of CO2 from shelf sea pumping. Science, 304: 1005–1008 Tseng C M, Liu K K, Gong G C, Shen P Y, Cai W J. 2011. CO2 uptake in the East China Sea relying on Changjiang runoff is prone to change.
Geophys Res Lett, 38: L24609
Tsunogai S, Watanabe S, Nakamura J, Ono T, Sato T. 1997. A preliminary study of carbon system in the East China Sea. J Oceanogr, 53: 9–17
Tsunogai S, Watanabe S, Sato T. 1999. Is there a “continental shelf pump” for the absorption of atmospheric CO2? Tellus B, 51: 701–712
Vitousek P M, Mooney H A, Lubchenco J, Melillo J M. 1997. Human domination of Earth’s ecosystems. Science, 277: 494–499
Walsh J J. 1991. Importance of continental margins in the marine bio- geochemical cycling of carbon and nitrogen. Nature, 350: 53–55
Wang S L, Arthur Chen C T, Hong G H, Chung C S. 2000. Carbon dioxide and related parameters in the East China Sea. Cont Shelf Res, 20: 525– 544
Wanninkhof R. 1992. Relationship between wind speed and gas exchange over the ocean. J Geophys Res, 97: 7373–7382
Wanninkhof R, Asher W E, Ho D T, Sweeney C, McGillis W R. 2009. Advances in quantifying air-sea gas exchange and environmental for- cing. Annu Rev Mar Sci, 1: 213–244
Xu X M, Zang K P, Zhao H D, Zheng N, Huo C, Wang J Y. 2016. Monthly CO2 at A4HDYD station in a productive shallow marginal sea (Yellow Sea) with a seasonal thermocline: Controlling processes. J Mar Syst, 159: 89–99
Xue L, Xue M, Zhang L J, Sun T, Guo Z, Wang J. 2012. Surface partial pressure of CO2 and air-sea exchange in the northern Yellow Sea. J Mar Syst, 105-108: 194–206
Xue L, Zhang L J, Cai W J, Jiang L Q. 2011. Air-sea CO2 fluxes in the southern Yellow Sea: An examination of the continental shelf pump hypothesis. Cont Shelf Res, 31: 1904–1914
Zhai W D, Dai M H, Guo X G. 2007. Carbonate system and CO2 degassing fluxes in the inner estuary of Changjiang (Yangtze) River, China. Mar Chem, 107: 342–356
Zhai W D, Dai M H. 2009. On the seasonal variation of air-sea CO2 fluxes in the outer Changjiang (Yangtze River) Estuary, East China Sea. Mar Chem, 117: 2–10
Zhai W D, Zang K P, Huo C, Zheng N, Xu X M. 2015. Occurrence of aragonite corrosive water in the North Yellow Sea, near the Yalu River estuary, during a summer flood. Estuar Coast Shelf Sci, 166: 199–208 Zhang L J, Xue L, Song M Q, Jiang C B. 2010. Distribution of the surface partial pressure of CO2 in the southern Yellow Sea and its controls. Cont
Shelf Res, 30: 293–304
Zhang Y H, Huang Z Q, Ma L M, Qiao R, Zhang B. 1997. Carbon dioxide in surface water and its flux in East China Sea (in Chinese with English abstract). J Oceanogr Taiwan Strait, 16: 37–42
(Responsible editor: Meixun ZHAO)