Effects of seagrass leaf litter decomposition on sediment organic carbon composition and the key transformation processes
LIU SongLin1-2, JIANG ZhiJian1, DENG YiQin3, WU YunChao1,2, ZHAO ChunYu1,2,
ZHANG JingPing1, SHEN Yuan1'2 & HUANG XiaoPing1"
1Key Laboratory of Tropical Marine Bio-resources and Ecology, South China Sea Institute of Oceanology, Chinese Academy of Sciences,
Guangzhou 510301, China;
2College of Earth Science, University of Chinese Academy of Sciences, Beijing 100049, China;
3South China Sea Fisheries Research Institute, Chinese Academy of Fishery Sciences, Guangzhou 510300, China
Received September 9, 2017; accepted November 16, 2017; published online November 21, 2017
Abstract Seagrass leaf litters are an important source of sediment organic carbon (SOC). However, the mechanisms of seagrass leaf litter decomposition influencing SOC composition and the key transformation processes remain unknown. We performed a laboratory chamber experiment to compare the labile organic carbon (OC) composition and the enzyme activities governing SOC transformation between the seagrass group (seagrass leaf litter addition) and the control group. The results showed that the seagrass leaf litter decomposition significantly elevated the salt-extractable carbon (SEC) content and the SEC/SOC. Additionally, the levels of invertase, polyphenol oxidase, and cellulase in the seagrass leaf litters addition group were generally higher than in the control group, which could elevate recalcitrant OC decomposition. Following 24 days incubation, addition of seagrass leaf litter increased the amount of CO2 released, but decreased the SOC content. Therefore, seagrass leaf litter decomposition leached abundant dissolved OC, which enhanced the activity and transformation of SOC.
Keywords Seagrass leaf litter, Sediment organic carbon, Composition, Enzyme activities
Citation: Liu S L, Jiang Z J, Deng Y Q, Wu Y C, Zhao C Y, Zhang J P, Shen Y, Huang X P. 2017. Effects of seagrass leaf litter decomposition on sediment organic
carbon composition and the key transformation processes. Science China Earth Sciences, 60: 2108-2117, https://doi.org/10.1007/s11430-017-9147-4
1.Introduction
Seagrass ecosystems are globally significant hotspots for organic carbon (OC) sequestration. Referred to as “blue carbon" these ecosystems store the majority of OC in sediments (Fourqurean etal., 2012; Duarte etal., 2013; Macreadie etal., 2014). It has been estimated that up to 19.9 Pg OC is stored in sediments of global seagrass meadows (Fourqurean et al., 2012). One of the important reasons for the high sediment OC (SOC) sequestration capacity of seagrasses meadows is its high primary productivity (Duarte et al., 2010). About 50% of seagrass primary production is allocated to the growth Corresponding author (email: xphuang@scsio.ac.cn) of aboveground biomass (Duarte and Chiscano, 1999), however, herbivores generally consume low amounts of seagrass leaves due to its unpalatability (Heck and Valentine, 2006). Most seagrass leaf production senesces and contributes to the detrital pool (Mateo et al., 2006). For example, 80% of Tha- lassia hemprichii leaf production flowed to detritus (Chiu et al., 2013). Therefore, seagrass leaf litter accumulation in seagrass meadows and the adjacent beaches is observed worldwide. (Coupland et al., 2007; Macreadie et al., 2017) (Figure 1). Three phases of seagrass decomposition are definable: leaching, microbial colonization, and slow breakdown of recalcitrant materials (Godshalk and Wetzel, 1978). The seagrass leaf litter contains abundant nonstructural carbohydrates, released as dissolved OC (DOC) into the water column or sediment during the initial leaching period (Vichkovit- ten and Holmer, 2004; Lavery et al., 2013). This can elevate microbial food webs (Lavery et al., 2013; Wang et al., 2014; Duarte, 2017) as well as microbial biomass and activity (Vichkovitten and Holmer, 2004; Fraser et al., 2016) in seagrass meadows and the adjacent coastal areas. In addition, transformation of SOC by sediment microorganisms is one of the determinants for SOC sequestration potential (Lopez et al., 1998; Holmer et al., 2004). Therefore, release of highly labile materials from seagrass leaf litter may influence the SOC compositions and transformation processes. Sediment labile OC (LOC) is a labile fraction of SOC that plays a crucial role in the SOC dynamics and regulates ecosystem productivity (Dodla et al., 2012). However, empirical evidence of sediment LOC dynamics in response to seagrass leaf litter decomposition is lacking.
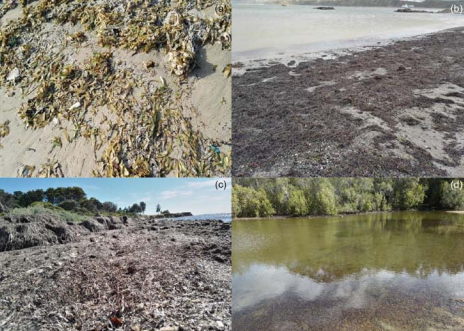
Sediment microbial extracellular enzymes are one of the active organic ingredients in sediment, and the enzymes governing OC transformation are closely related to SOC decomposition (Karaca et al., 2011). The response of sediment microbial extracellular enzyme activities to environment and sediment organic matter changes rapidly (Zhang et al., 2014; Shao et al., 2015), with important implications for perceptions of SOC sequestration potential (Liu et al., 2017a, 2017b, 2017c). Nevertheless, the effects of seagrass leaf litter decomposition on enzymatic activities that influence SOC transformation remain unknown.
The seagrass meadows of Xincun Bay, Hainan Island, China, are located in a nearly closed bay and contain the typical tropical seagrass ecosystems, with abundant seagrass diversity. Large amounts of seagrass leaf litter were observed in the intertidal areas of Xincun Bay (Figure 1a). In this study, we used laboratory incubation procedures to compare the differences in salt-extractable carbon (SEC), microbial biomass carbon (MBC) contents, and enzyme activities of polyphenol oxidase, dehydrogenase, invertase, and p-glucosidase between the seagrass group (with addition of senescent seagrass) and a control group (without addition of senescent seagrass). MBC is a measure of microbial biomass and a vital fraction of LOC, whereas SEC is an immediate source of carbon for sediment microbial metabolism (Rochette and Gregorich, 1998; Liu et al., 2016). In addition, the activities of polyphenol oxidase, dehydrogenase, invertase, and p-glucosidase are positively correlated with SOC transformation (Waldrop et al., 2004; Yin et al., 2014; Shao et al., 2014; Liu et al., 2017a, 2017b, 2017c). Our goal was to generate empirical data that could help understand the SOC transformation process in response to seagrass leaf litter decomposition, thereby providing important scientific evidence for blue carbon management and protection within seagrass meadows.
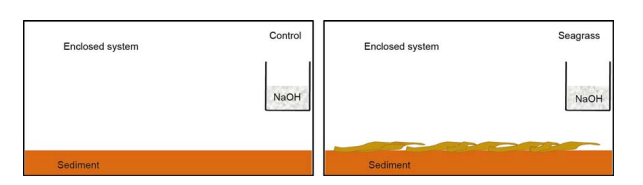
Figure 2 Schematic diagram for the experimental setup
2.Materials and methods
2.1 Sample collection
The surface sediment (0-1 cm) and old leaf blades still attached to Thalassia hemprichii shoots were collected during low tides in December 2016 at Xincun Bay, Hainan Island, China. Following collection, the seagrass leaves and sediment samples were stored in an ice chest and transported to the laboratory within a few hours. In addition, seagrass leaf litter biomass was determined by calculating seagrass leaf litter weight across ten randomly sampled quadrats (25 cm x 25 cm) in Xincun Bay.
2.2Experimental setup
The sediments were air-dried and then sieved through a 35-mesh (500 pm) screen. The old seagrass leaves were rinsed with Milli-Q water, and some seagrass and sediment samples were set aside for the initial OC content measurements.
Five hundred grams of the sediment (dry weight, DW) were placed into acid-washed 27 cmx 17 cmx 25 cm glass jars (n=24), resulting in a 1-cm sediment height. Then 250 mL of30 ppt artificial seawater (in situ seawater salinity) was poured into each glass jar. The incubation experiment was divided into a seagrass group (with seagrass addition) and a control group (without seagrass addition) (Figure 2). To generate the seagrass addition treatment, we added 20 g wet weight of old seagrass leaves in half of the glass jars, but the control group did not contain additional seagrass. In addition, a beaker filled with 120 mL 0.1 mol dm-3 NaOH was placed in each glass jar for CO2 trapping. All glass jars were sealed during incubation in the dark. The first 3-4 weeks were the leaching period of seagrass leaf litter decomposition (Kristensen, 1994; Wang et al., 2014). At 0, 2, 5, 13, and 24 days, we removed three glass jars of each treatment to measure the amount of CO2 released and parameters of seagrass and sediment analysis.
2.3Sample analysis
At each sampling time point, the beaker filled with NaOH was immediately sealed, and we then measured the oxidation reduction potential of the sediment. All seagrass samples were removed, rinsed with Milli-Q water, and then dried at 60 C to a constant weight to calculate the remaining biomass. Seagrass samples were ground to a fine powder, filtered through a 100-mesh screen, and stored in a desiccator. The sediment sample was divided into three sub-samples; one was air-dried and the other two were stored at either -20 C or 4 C.
To measure the amount of CO2 released during incubation, we used an alkali absorption method. Briefly, was extracted to a conical flask, and then 2.5 mL 1 mol L-1 BaCk and two drops of phenolphthalein were added. This solution was then titrated with 1 mol L-1 HCl until the red color disappeared (Nunez et al., 2001). Sediment pH was measured at a ratio of 1:2.5 sediment:water suspension using a Seven 2Go? pH Meter (Liu et al., 2017a).
The sediment stored at 4 C was used to measure sediment enzyme activities (polyphenol oxidase, dehydrogenase, invertase, and p-glucosidase), SEC, and MBC. Polyphenol oxidase activity was determined by spectrophotometry of pyrogallic acid. Following incubation at 30 C for 2 h, the purpurogallin produced was extracted with ether and then measured at 430 nm. Polyphenol oxidase activity was determined by the amount of purpurogallin (mg) produced by per gram dry weight sediment within 2 h (Burns and Dick, 2002; Yin et al., 2014). Invertase activity was determined by spectrophotometry of 3,5-dinitrosalicylic acid colorimetry. After incubation at 37 C for 24 h, the product of invertase was glucose, which was measured at 550 nm. Invertase activity was determined by the amount of glucose produced (mg) per gram dry weight sediment within 24 h (Yin et al., 2014; Shao et al., 2015). Dehydrogenase activity was measured using triphenyl tetrazolium chloride as a substrate. After incubation at 37 C for 24 h, the amount of triphenyl formazan was measured at 485 nm. Sediment dehydrogenase activity was expressed as pg triphenyl formazan g-1 dry sediment 24 h-1 (Wei-xiang et al, 2004). p-glucosidase activity was measured using Q-nitrophenyl-p-d-glucopyra- noside as a substrate. After incubation at 37 C for 1 h, the amount of Q-nitrophenol was measured at 410 nm. The p-glucosidase activity was expressed as pmol Q-nitrophenol g-1 dry sediment 1 h-1 (Yin et al., 2014; Shao et al., 2015). MBC was measured by fumigating 10 g moist sediment in an ethanol-free chloroform atmosphere for 24 h in a 25 C dark room, which was quickly extracted with 0.5 M K2SO4.
Meanwhile, another unfumigated 10 g moist sediment was extracted with 0.5 M K2SO4. The mixture was shaken for 30 min and then centrifuged for 10 min; at this point, the supernatant was vacuum-filtered through pre-combusted GF/F filters (Whatman, 450 C, 3 h) into a pre-combusted apragaz bottle (450 C, 3 h) for total OC analysis. The filtrates for the fumigation-treated and untreated sediments were determined on a Shimadzu TOC analyzer (TOC-Vcph). The OC contents of filtrate extracted from unfumigated sediment represented SEC (Fang et al., 2005; Dodla et al., 2012), whereas MBC was calculated according to the equation: MBC= (OCfumigated_SEC)/0.38 (Vance etal., 1987; Yang et al., 2013).
The frozen sediment was freeze-dried, ground, and homogenized using a mortar and pestle and filtered through a 100- mesh screen; at this point, it was acidified (in 1 mol L_1 HCl) to remove carbonate and then washed with distilled water before drying at 40 C in an oven. We used an elemental analyzer (Vario EL) to determine the SOC and seagrass OC contents. The OC differences between the initial seagrass sample and each of the sampling points represented the amount at which OC was released over time.
2.4 Data analysis
Data were log- or exponent-transformed, ifnecessary, to meet the assumption of homogeneity of variance. At each sampling time, sediment oxidation reduction potential, sediment pH, SOC, SEC, MBC, SEC/SOC, MBC/SOC, polyphenol oxidase, dehydrogenase, invertase, and p-glucosidase were compared between the seagrass and control groups using Student's /-test. To describe the OC transformation process of the incubation system during the 24 days experiment, we calculated the net variation of the seagrass OC, SEC, MBC, SOC, CO2, and the abovementioned four enzyme activity levels between the initial time point and after the 24 days incubation. The formula Ket=^24d-K)d was used to determine variation of the above parameters. Fnet indicated the net variation amount, whereas Vm and V4d indicated either the initial and after 24 days incubation parameters, respectively. If not mentioned the release or assimilation, negative and positive numbers represented the decrease and increase, respectively.
3.Results
3.1 OC released from seagrass leaf litter
During 24 days incubation, 254.42 mg (average value) OC was released from seagrass leaf litter (Figure 3a), which accounted for <20% of the total OC of seagrass leaf litter (Figure 3b).
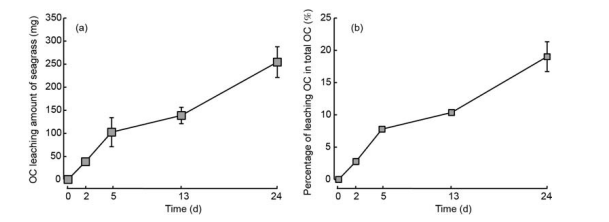
Figure 3 Amount of leaching of OC (a), and the percentage of OC leaching from seagrass leaf litter (b).
3.2Variation in sediment physical indicators
The sediment oxidation reduction potentials of the seagrass and control groups presented the trend of first to decrease with increase in incubation time, with the lowest value observed on day 5 (Figure 4a). At each sampling time after 0 day, the sediment oxidation reduction potential of the control group was significantly higher than the seagrass group (p<0.05). Additionally, the sediment pH showed a similar trend with sediment oxidation reduction potential, with the lowest value observed on day 2. There was no significant difference of sediment pH between the seagrass and control groups at each sampling time (Figure 4b, ^>0.05).
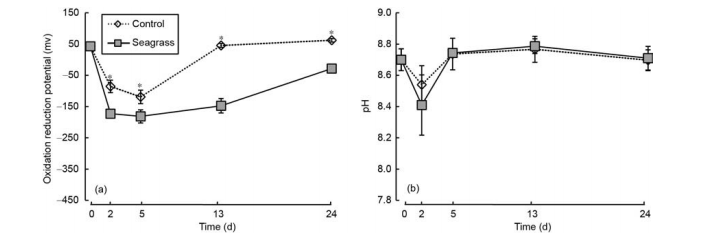
Figure 4 Sediment oxidation reduction potential (a) and pH (b) in the seagrass and control groups. The asterisks above bars indicate significant difference between the two groups within the same sampling time (Student's t-test, ?<0.05).
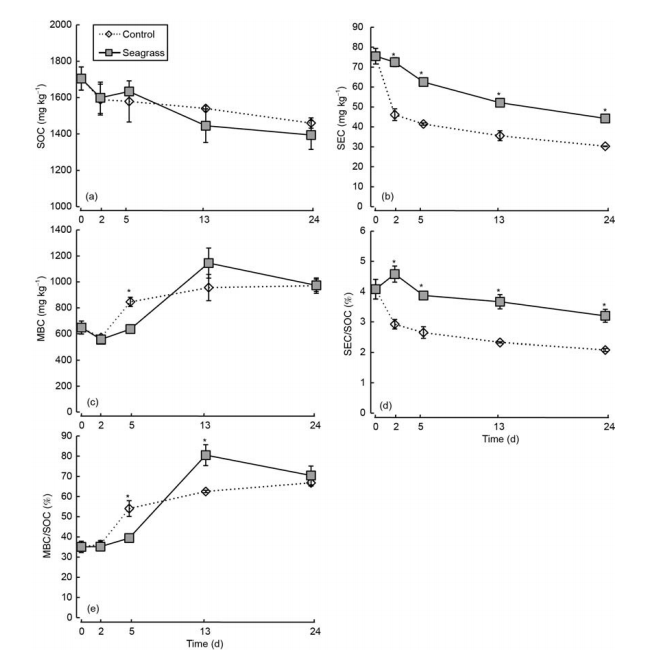
Figure 5 SOC (a), SEC (b), MBC (c), SEC/SOC (d), and MBC/SOC (e) in the seagrass and control groups. The asterisks above the bars indicate significant differences between the two groups within the same sampling time (Student's t-test, p<0.05).
3.3Variation in SOC composition
SOC, SEC, and SEC/SOC generally showed gradually decreasing trends, both in the seagrass and control groups (Figure 5a, b, and c). SEC and SEC/SOC of the seagrass group were higher than the control group during 2-24 days (Figure 5b and c, q<0.05), whereas SOC was not significantly different between the two groups (Figure 5a, ^>0.05). In addition, MBC and MBC/SOC first decreased and then increased before becoming stable (Figure 5c and e). MBC and MBC/SOC were much higher in the control group than the seagrass group on day 5 (Figure 5c and e, q<0.05), but these values in the seagrass group were higher than the control group following longer incubation periods (Figure 5c and e).
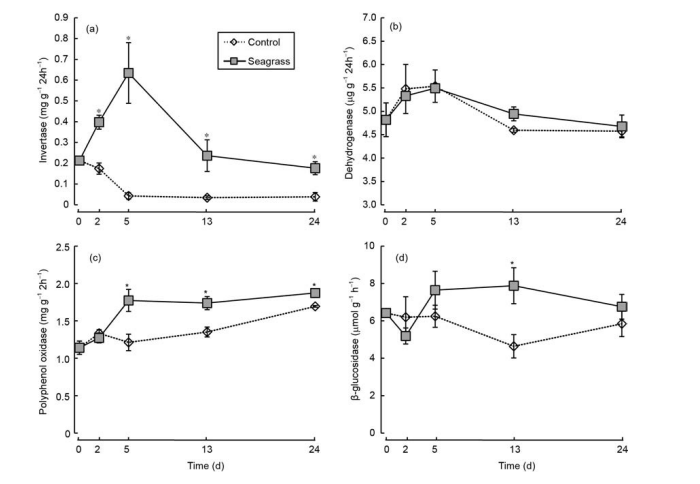
Figure 6 Sediment invertase (a), dehydrogenase (b), polyphenol oxidase (c), and p-glucosidase (d) activities in the seagrass and control groups. The asterisks above bars indicate significant difference between the two groups within the same sampling time (Student's t-test, p<0.05).
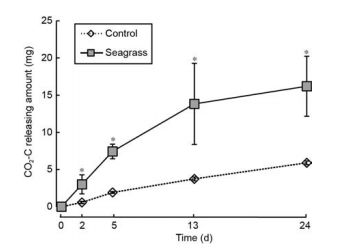
Figure 7 The amount of CO2 released in the seagrass and control groups. The asterisks above the bars indicate significant difference between the two groups within the same sampling time (Student's t-test, p< 0.05).
3.4Variation in sediment enzymes activities
The amount of OC released increased with incubation time.
Invertase activity of the seagrass group initially increased and then decreased, with the highest value appearing on day 5. By contrast, invertase activity of the control group initially decreased and then stabilized (Figure 6a). A significantly higher invertase activity was observed in the seagrass group than in the control group during days 2-24 (Figure 6a, ^<0.05). There were no significant differences in dehydrogenase activities, which initially increased and then decreased, between the seagrass and control groups (p>0.05), with the highest value observed on day 5 (Figure 6b). Polyphenol oxidase activities increased with incubation time, and the polyphenol oxidase activities of the seagrass group were significantly higher than the control group after 5 days (Figure 6c, q<0.05). Additionally, the p-glucosidase activity of the seagrass group was higher than the control group after 5 days and was markedly different on day 13 (Figure 6d, p<0.05).
3.5 Levels of CO2 release in the incubation system
We used carbon contents to represent the amount of CO2 released (CO2-C) in the incubation system (Figure 7). CO2-C levels increased with incubation time for both groups. After 24 days incubation, the average amount of CO2-C released in the control and seagrass groups were 5.90 and 16.20 mg, respectively.
4.Discussion
4.1 Responses of SOC composition and enzymes activities to seagrass leaf litter decomposition
Seagrass leaf litter decomposition occurs in different phases, with large amounts of nonstructural carbohydrates and amino acids released during the initial leaching period (Maie et al., 2006; Lavery et al., 2013). The relative contents of lignin and cellulose increased during decomposition, which decreased the rate of seagrass leaf litter decomposition (Mateo et al., 2006). The initial leaching period lasted for 24 days in this study; at this point, an abundant amount of DOC was released and resulted in rapid transformation by microorganisms (Kristensen, 1994; Wang et al., 2014). MBC of both the groups decreased during the first two days of incubation due to their acclimation to the new environment, but then it increased. However, leaching of DOC from seagrass leaf litter occurred the fastest during the first five days of incubation (Pedersen et al., 1999; Zhou et al., 2014), although large amounts of DOC inputs could retard sediment microbial growth and activity in the short term (Kristensen et al., 1995; Kristensen and Hansen, 1995). Therefore, MBC and MBC/SOC of the seagrass group were significantly lower than the control group. In addition, SEC and SEC/SOC showed a decreasing trend, which could be attributed to the susceptibility of SEC to microbial degradation (Yang et al., 2013; Liu et al., 2016). Furthermore, abundant DOC leaching from seagrass leaf litter, including nonstructural carbohydrates (sucrose, mannose, xylose et al.), amino acids, and polyphenol, became the important components of SEC (Vichkovitten and Holmer, 2004; Maie et al., 2006; Lavery et al., 2013), which led to significantly higher SEC and SEC/SOC in the seagrass group than in the control group. Meanwhile, high SEC contents could induce high microbial growth and activity (Kristensen et al., 1995), which could enhance MBC and MBC/SOC of the seagrass group during the later periods of incubation.
The sediment enzymes activities are correlated with microbial secretion and reaction substrate concentration (Zhang et al., 2011; Shao et al., 2015; Liu et al., 2017a). Except dehydrogenase, the enzyme activities in the control group were lower than in the seagrass group. High microbial biomass, abundant sucrose, and polyphenol leaching from seagrass leaf litter in the seagrass group showed significantly elevated activities of invertase and polyphenol oxidase (Stemmer et al., 1998; Shao et al., 2015; Liu et al., 2017a, 2017b). Additionally, the p-glucosidase activities of the seagrass group generally were higher than the control group, which may be due to the abundant cellulose in the seagrass leaf litter (DeForest, 2009). Invertase catalyzes sucrose hydrolysis, with releasing glucose and fructose as highly active metabolic compounds that can be used directly by microorganisms (Stemmer et al., 1998). The seagrass leaf litter rapidly released large amounts of DOC during the first five days, which induced invertase activities to increase sharply, with the highest invertase activity observed on day 5. However, invertase activities decreased after five days of incubation, likely due to the DOC leaching rates of seagrass leaf litter (Lavery et al., 2013; Zhou et al., 2014) and decreases in SEC content with incubation time (Yang et al., 2013; Liu et al., 2016). Sediment recalcitrant materials mainly comprised lignin and cellulose (Mateo et al., 2006), which can be degraded by polyphenol oxidase and p-glucosidase, respectively (DeForest et al., 2004; Waldrop et al., 2004; DeForest, 2009). Therefore, the high polyphenol oxidase and p-glucosidase activities possibly enhanced sediment recalcitrant OC transformation. This did not benefite the SOC sequestration (Liu et al., 2017a, 2017c). In addition, polyphenol oxidase and p-glucosidase activities of the control group also increased with incubation time, ascribed to the decline of SEC, thereby accelerating sediment recalcitrant OC transformation. Furthermore, polyphenol oxidase can stimulate hydrolytic enzymes activities responsible for SOC decomposition by eliminating phenolic compound contents (Freeman et al., 2001). Dehydrogenase represented the sediment microbial activities and intensity of organic matter decomposition (Wei-xiang et al., 2004). Dehyrdrogenase activities increased during the first five days due to inceases in microbial biomass and abundant LOC in sediment, but then decreases in SEC content and the anaerobic environment constrained the dehydrogenase activities (Ying et al., 2007).
4.2 Net balance of SOC
Due to variations in seagrass OC, SEC, MBC, SOC, and activities of the four enzymes between the initial and after 24 days incubation, we plotted the OC transformation paths of the seagrass and control groups (Figure 8). During 24 days incubation, SOC contents of the seagrass and control groups decreased due to microbial transformation. There were no OC inputs in the sediment of the control group; therefore, microbes transformed SOC, resulting in a decline of SOC content. Nevertheless, the sediment of the seagrass group contained OC inputs, which led to more SOC loss, a result that is consistent with a study by Holmer and Olsen (2002). In the seagrass group, seagrass leaf litter leached abundant DOC during the 24 days incubation, which elevated the microbial biomass and released extracellular enzymes. As a consequence of elevated activities of invertase, polyphenol oxidase, and p-glucosidase due to seagrass leaf litter decomposition, there was a rapid transformation of SOC and a large of amount of CO2 was released. This could explain the greater reduction of SOC in the seagrass group than in the control group. After the 24 days incubation, the remaining seagrass OC accounted for 80% of the total seagrass OC, with a majority of OC being recalcitrant OC, such as lignin and cellulose (Mateo et al., 2006; Lavery et al., 2013), which can become the important constituents of SOC storage (Cebrian, 2002; Macreadie et al., 2017). However, in this study, we only considered the effects of seagrass leaf litter leaching on SOC transformation; the effects of long-term decomposition of seagrass leaf litter on SOC net balance requires further examination.
Seagrass OC and SOC loss during incubation was generally higher than the amount of CO2 recovered (Figure 8). The deficit in OC recovery may be due to several factors. First, OC loss may have been due to seagrass leaf litter recovery, and CO2 may have dissolved in the sediment porewater. Second, carbonate precipitation due to decomposition may have affected sediment pH. However, the pH did slightly change during incubation, suggesting that this effect would have been minor (Mucci et al., 2000). Third, methane also would have been produced due to SOC decomposition (Gonsalves et al., 2011), however, we did not measure in this study. Finally, during the leaching period, LOC released from seagrass leaf litter would have rapidly transformed by the seagrass leaf litter surface microbes (Robertson et al., 1982; Blum and Mills, 1991; Peduzzi and Herndl, 1991). However, the seagrass leaf litter was washed after collection, which would have removed majority of the MBC attached to the seagrass leaf litter surface.
4.3 Ecological implications
Seagrass ecosystems can produce large amounts of seagrass wrack, which provides many important ecological functions, including important food sources and habitats for seabirds andzoobenthos (Dugan et al., 2003) and sources of neighboring ecosystems' sediment and nutrients (Jimenez et al., 2017). Seagrass leaf litter carbon budgets are gaining attention due to more studies on the function of blue carbon seagrass meadows (Chiu et al., 2013; Jimenez et al., 2017; Macreadie et al., 2017; Misson et al., 2017). Indeed, seagrass leaf litter decomposition would produce CO2 as a carbon source of coastal habitats (Coupland et al., 2007; Misson et al., 2017). However, the recalcitrant OC of seagrass leaf litter could be an important source of OC storage for coastal habitats (Macreadie et al., 2017). In this study, seagrass leaf litter decomposition released large amounts of DOC and elevated the labile composition of SOC and microbial extracellular enzymes activities, which stimulated the SOC transformation and consequently increased SOC loss. In addition, eutrophication is a key driving factor of global seagrass meadows decline (Green and Short, 2003). With increase in global nutrient load, the nutrient-induced loss of seagrass could decrease the OC storage in living seagrass biomass (Russell et al., 2013). In addition, eutrophication could elevate seagrass leaf litter production and amounts of DOC released from seagrass leaf litter (Zhou et al., 2014). Therefore, increases in the nutrient-induced seagrass leaf litter biomass could accelerate the SOC transformation and decrease the sequestration of OC in seagrass meadows, which would further affect the global climate.
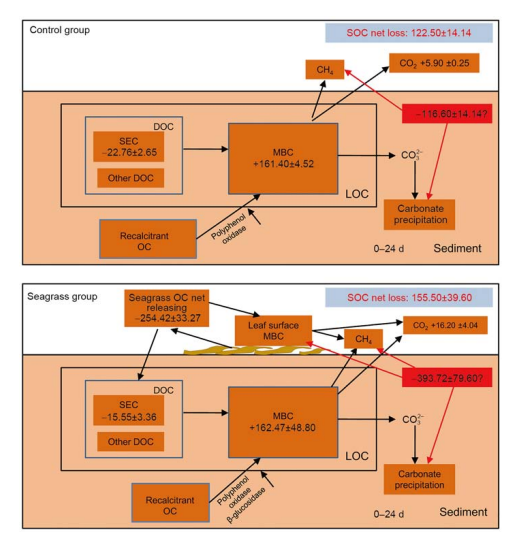
Figure 7 The amount of CO2 released in the seagrass and control groups. The asterisks above the bars indicate significant difference between the two groups within the same sampling time (Student's t-test, p< 0.05).
5. Conclusion
The seagrass leaf litter decomposition significantly elevated the LOC content and contribution of LOC in SOC as well as stimulated enzyme activities related to SOC decomposition, which enhanced the amount of CO2 released and reduced the SOC content. These results indicated that seagrass leaf litter decomposition could increase the LOC pool in SOC and elevate SOC transformation.
Acknowledgements This work was supported by the National Basic Research Program ofChina (GrantNos. 2015CB452905, 2015CB452902), the National Natural Science Foundation of China (Grant Nos. 41730529, 41306108, 41406128), and the National Specialized Project of Science and Technology (GrantNo. 2015FY110600).
References
Blum L, Mills A. 1991. Microbial growth and activity during the initial stages of seagrass decomposition. Mar Ecol Prog Ser, 70: 73-82
Burns R G, Dick R P. 2002. Enzymes in the environment: Activity, ecology, and applications. CRC Press
Cebrian J. 2002. Variability and control of carbon consumption, export, and accumulation in marine communities. Limnol Oceanogr, 47: 11-22
Chiu S H, Huang Y H, Lin H J. 2013. Carbon budget of leaves of the tropical intertidal seagrass Thalassia hemprichii. Estuarine Coastal Shelf Sci, 125: 27-35
Coupland G T, Duarte C M, Walker D I. 2007. High metabolic rates in beach cast communities. Ecosystems, 10: 1341-1350
DeForest J L. 2009. The influence of time, storage temperature, and substrate age on potential soil enzyme activity in acidic forest soils using MUB- linked substrates and l-DOPA. Soil Biol Biochem, 41: 1180-1186
DeForest J L, Zak D R, Pregitzer K S, Burton A J. 2004. Atmospheric nitrate deposition, microbial community composition, and enzyme activity in Northern Hardwood forests. Soil Sci Soc Am J, 68: 132-138
Dodla S K, Wang J J, Delaune R D. 2012. Characterization of labile organic carbon in coastal wetland soils of the Mississippi River deltaic plain: Relationships to carbon functionalities. Sci Total Environ, 435436: 151-158
Duarte C M. 2017. Reviews and syntheses: Hidden forests, the role of vegetated coastal habitats in the ocean carbon budget. Biogeosciences, 14: 301-310
Duarte C M, Chiscano C L. 1999. Seagrass biomass and production: A reassessment. Aquatic Bot, 65: 159-174
Duarte C M, Kennedy H, Marba N, Hendriks I. 2013. Assessing the capacity of seagrass meadows for carbon burial: Current limitations and future strategies. Ocean Coastal Manage, 83: 32-38
Duarte C M, Marba N, Gacia E, Fourqurean J W, Beggins J, Barron C, Apostolaki E T. 2010. Seagrass community metabolism: Assessing the carbon sink capacity of seagrass meadows. glob Biogeochem Cycle, 24: GB4032
Dugan J E, Hubbard D M, McCrary M D, Pierson M O. 2003. The response
of macrofauna communities and shorebirds to macrophyte wrack subsidies on exposed sandy beaches of southern California. Estuarine Coastal Shelf Sci, 58: 25-40
Fang C, Smith R Moncrieff JB, Smith JU. 2005. Erratum: Similar response of labile and resistant soil organic matter pools to changes in temperature. Nature, 436: 881-881
Fourqurean J W, Duarte C M, Kennedy H, Marba N, Holmer M, Mateo M A, Apostolaki E T, Kendrick G A, Krause-Jensen D, McGlathery K J, Serrano O. 2012. Seagrass ecosystems as a globally significant carbon stock. Nat Geosci, 5: 505-509
Fraser M W, Statton J, Hovey R K, Laverock B, Kendrick G A. 2016. Seagrass derived organic matter influences biogeochemistry, microbial communities, and seedling biomass partitioning in seagrass sediments. Plant Soil, 400: 133-146
Freeman C, Ostle N, Kang H. 2001. An enzymic 'latch' on a global carbon store. Nature, 409: 149
Godshalk G L, Wetzel R G. 1978. Decomposition of aquatic angiosperms. III. Zostera marina L. and a conceptual model of decomposition. Aquat Bot, 5: 329-354
Gonsalves M J, Fernandes C E G, Fernandes S O, Kirchman D L, Bharathi P A L. 2011. Effects of composition of labile organic matter on biogenic production of methane in the coastal sediments of the Arabian Sea. Environ Monit Assess, 182: 385-395
Green E P, Short F T. 2003. World Atlas of Seagrasses. Berkeley: University of California Press
Heck Jr. K L, Valentine J F. 2006. Plant-herbivore interactions in seagrass meadows. J Exp Mar Biol Ecol, 330: 420-436
Holmer M, Duarte C, Boschker H, Barron C. 2004. Carbon cycling and bacterial carbon sources in pristine and impacted Mediterranean seagrass sediments. Aquat Microb Ecol, 36: 227-237
Holmer M, Olsen A B. 2002. Role of decomposition of mangrove and seagrass detritus in sediment carbon and nitrogen cycling in a tropical mangrove forest. Mar Ecol Prog Ser, 230: 87-101
Jimenez M A, Beltran R, Traveset A, Calleja M L, Delgado-Huertas A, Marba N. 2017. Aeolian transport of seagrass (Posidonia oceanica) beach-cast to terrestrial systems. Estuar Coast Shelf Sci, 196: 31-44
Karaca A, Cetin S C, Turgay O C, Kizilkaya R. 2011. Soil enzymes as indication of soil quality. In: Shukla G, Varma A, eds. Soil Enzymology. Heidelberg: Springer-Verlag, Berlin. 119-148
Kristensen E. 1994. Decomposition of macroalgae, vascular plants and sediment detritus in seawater: Use of stepwise thermogravimetry. Biogeochemistry, 26: 1-24
Kristensen E, Ahmed S I, Devol A H. 1995. Aerobic and anaerobic decomposition of organic matter in marine sediment: Which is fastest? Limnol Oceanogr, 40: 1430-1437
Kristensen E, Hansen K. 1995. Decay of plant detritus in organic-poor marine sediment: Production rates and stoichiometry of dissolved C and N compounds. Issn-0022-2402, 53: 675-702
Lopez N I, Duarte C M, Vallespinos F, Romero J, Alcoverro T. 1998. The effect of nutrient additions on bacterial activity in seagrass (Posidonia oceanica) sediments. J Exp Mar Biol Ecol, 224: 155-166
Lavery P, McMahon K, Weyers J, Boyce M, Oldham C. 2013. Release of dissolved organic carbon from seagrass wrack and its implications for trophic connectivity. Mar Ecol Prog Ser, 494: 121-133
Liu S, Jiang Z, Wu Y, Zhang J, Arbi I, Ye F, Huang X, Macreadie P I. 2017a. Effects of nutrient load on microbial activities within a seagrass-dominated ecosystem: Implications of changes in seagrass blue carbon. Mar Pollut Bull, 117: 214-221
Liu S, Jiang Z, Zhang J, Gan M, Wu Y, Huang X. 2017b. Characteristics of key enzyme activities influencing sediment organic carbon transformation and their response to the nutrient loading in seagrass bed of Xincun bay,Hainan Island (in Chinese). Mar Environ Sci, 36: 1-7
Liu S, Jiang Z, Zhang J, Wu Y, Huang X, Macreadie P I. 2017c. Sediment microbes mediate the impact of nutrient loading on blue carbon
sequestration by mixed seagrass meadows. Sci Total Environ, 599-600: 1479-1484
Liu S, Jiang Z, Zhang J, Wu Y, Lian Z, Huang X. 2016. Effect of nutrient enrichment on the source and composition of sediment organic carbon in tropical seagrass beds in the South China Sea. Mar Pollut Bull, 110: 274-280
Macreadie P I, Baird M E, Trevathan-Tackett S M, Larkum A W D, Ralph P J. 2014. Quantifying and modelling the carbon sequestration capacity of seagrass meadows一A critical assessment. MarPollutBull, 83: 430X39
Macreadie P I, Trevathan-Tackett S M, Baldock J A, Kelleway J J. 2017. Converting beach-cast seagrass wrack into biochar: A climate-friendly solution to a coastal problem. Sci Total Environ, 574: 90-94
Maie N, Jaffe R, Miyoshi T, Childers D L. 2006. Quantitative and qualitative aspects of dissolved organic carbon leached from senescent plants in an oligotrophic Wetland. Biogeochemistry, 78: 285-314
Mateo M A, Cebrian J, Dunton K, Mutchler T. 2006. Carbon flux in seagrass ecosystems. In: Larkum, A W D, Orth R J, Duarte C M, eds. Seagrasses: Biology, ecology and conservation. Springer, Dordrecht. 159-192
Misson G, Incerti G, Alberti G, Delle Vedove G, Pirelli T, Peressotti A. 2017. Assessing the contribution of beach-cast seagrass wrack to global GHGs emissions: Experimental models, problems and perspectives. EGU General Assembly Conference Abstracts. 19416
Mucci A, Sundby B, Gehlen M, Arakaki T, Zhong S, Silverberg N. 2000. The fate of carbon in continental shelf sediments of eastern Canada: A case study. Deep Sea Res Part II-Topic Stud Oceanogr, 47: 733-760
Nunez S, Martinez-Yrizar A, Burquez A, Garcia-Oliva F. 2001. Carbon mineralization in the southern Sonoran Desert. Acta Oecol, 22: 269-276
Pedersen A G U, Berntsen J, Lomstein B A. 1999. The effect of eelgrass decomposition on sediment carbon and nitrogen cycling: A controlled laboratory experiment. Limnol Oceanogr, 44: 1978-1992
Peduzzi P, Herndl G. 1991. Decomposition andsignificance of sea-grass leaf litter (Cymodocea nodosa) for the microbial food web in coastal waters (Gulf of Trieste, Northern Adriatic Sea). Mar Ecol Prog Ser, 71: 163-174 Robertson M, Mills A, Zieman J. 1982. Microbial synthesis of detrituslike particulates from dissolved organic carbon released by tropical seagrasses. Mar Ecol Prog Ser, 7: 279-285
Rochette P, Gregorich E G. 1998. Dynamics of soil microbial biomass C, soluble organic C and CO2 evolution after three years of manure application. Can J Soil Sci, 78: 283-290
Russell B D, Connell S D, Uthicke S, Muehllehner N, Fabricius K E, HallSpencer J M. 2013. Future seagrass beds: Can increased productivity lead to increased carbon storage? Mar Pollut Bull, 73: 463X69
Shao X, Yang W, Wu M. 2015. Seasonal dynamics of soil labile organic carbon and enzyme activities in relation to vegetation types in Hangzhou Bay tidal flat wetland. PLoS ONE, 10: e0142677
Stemmer M, Gerzabek M H, Kandeler E. 1998. Organic matter and enzyme activity in particle-size fractions of soils obtained after low-energy sonication. Soil Biol Biochem, 30: 9-17
Vance E D, Brookes P C, Jenkinson D S. 1987. An extraction method for measuring soil microbial biomass C. Soil Biol Biochem, 19: 703-707
Vichkovitten T, Holmer M. 2004. Contribution of plant carbohydrates to sedimentary carbon mineralization. Org Geo Chem, 35: 1053-1066
Waldrop M P, Zak D R, Sinsabaugh R L. 2004. Microbial community response to nitrogen deposition in northern forest ecosystems. Soil Biol Biochem, 36: 1443-1451
Wang X, Chen R F, Cable J E, Cherrier J. 2014. Leaching and microbial degradation of dissolved organic matter from salt marsh plants and seagrasses. Aquat Sci, 76: 595-609
Wei-xiang W, Qing-fu Y, Hang M, Xue-jun D, Wen-ming J. 2004. Bt-trans- genic rice straw affects the culturable microbiota and dehydrogenase and phosphatase activities in a flooded paddy soil. Soil Biol Biochem, 36: 289-295
Yang W, Zhao H, Chen X, Yin S, Cheng X, An S. 2013. Consequences of short-term C4 plant Spartina alterniflora invasions for soil organic carbon dynamics in a coastal wetland of Eastern China. Ecol Eng, 61: 50-57
Yin R, Deng H, Wang H, Zhang B. 2014. Vegetation type affects soil enzyme activities and microbial functional diversity following re-vegetation of a severely eroded red soil in sub-tropical China. CATENA, 115: 96-103
Ying G G, Yu X Y, Kookana R S. 2007. Biological degradation of triclo- carban and triclosan in a soil under aerobic and anaerobic conditions and comparison with environmental fate modelling. Environ Pollut, 150: 300-305
Zhang C, Liu G, Xue S, Song Z. 2011. Rhizosphere soil microbial activity under different vegetation types on the Loess Plateau, China. Geoderma, 161: 115-125
Zhang L, Song L, Shao H, Shao C, Li M, Liu M, Brestic M, Xu G. 2014. Spatio-temporal variation of rhizosphere soil microbial abundance and enzyme activities under different vegetation types in the coastal zone, Shandong, China. Plant BioSyst-An Int J Deal all Aspects Plant Biol, 148: 403-409
Zhou C, Jiang Z, Lian Z, Zhang J, Ni Z, Xu B, Huang X. 2014. Characteristics of seagrass Thalassia hemprichii leaf litter and its response to the fish farming in the Xincun Bay, Hainan Island (in Chinese). Chin J Ecol, 33: 1546-1552