Climate tipping-point potential and paradoxical production of methane in a changing ocean
Hongyue DANG & Jia LI2
1 State Key Laboratory of Marine Environmental Science, Institute of Marine Microbes and Ecospheres, College of Ocean and Earth Sciences,
Xiamen University, Xiamen 361102, China;
2 Tianjin Branch of China National Offshore Oil Corporation Ltd Tianjin 300452, China
Received October 31, 2017; revised April 11, 2018; accepted September 31, 2018; published online October 31, 2018
Abstract The global warming potential of methane (CH4) is about 30 times stronger than that of carbon dioxide (CO2) over a century timescale. Methane emission is hypothesized to have contributed to global climate change events and mass extinctions during Earth's history. Therefore, the study of CH4 production processes is critically important to the understanding of global climate change. It has been a dogma that biogenic CH4 detectable in the oceans originates exclusively from the anaerobic metabolic activity of methanogenic archaea in hypoxic and anoxic environments, despite reports that many oxic surface and near-surface waters of the world's oceans are CH4-supersaturated, thereby rendering net sea-to-air emissions of CH4. The phenomenon of CH4 production in oxic marine waters is referred to as the “ocean methane paradox”. Although still not totally resolved, recent studies have generated several hypotheses regarding the sources of CH4 production in oxic seawater. This review will summarize our current understanding of the importance of CH4 in the global climate and analyze the biological processes and their underpinning mechanisms that lead to the production of CH4 in oxic seawater environments. We will also tentatively explore the relationships of these microbial metabolic processes with global changes in climate and environment.
Keywords Ocean methane paradox, Gas hydrate, Marine particles, Methylphosphonate, DSMP, Global warming, Ocean deoxygenation, Ocean acidification
Citation: Dang H, Li J. 2018. Climate tipping-point potential and paradoxical production of methane in a changing ocean. Science China Earth Sciences, 61: 1714-1727, https://doi.org/lQ.lQQ7/sll43Q-Q17-9265-y
1.Introduction
Global warming, caused mainly by the rapid increase of anthropogenic greenhouse gas emissions, is dramatically changing the Earth's climate and environment. In May 2013, for the first time in human history, the daily average atmospheric carbon dioxide (CO2) concentration exceeded 400 ppm (parts per million), as measured at the Mauna Loa Observatory, Hawaii (Showstack, 2013). This atmospheric CO2 concentration is likely to be the highest during the last3.4Myr, 15 Myr, or even 34 Myr of the Earth's history (Beerling and Royer, 2011; Bijma et al., 2013; Brigham- Grette et al., 2013). The current anthropogenic carbon release rate (?10 Pg C yr 1) is nearly 10 times larger than that of the Palaeocene-Eocene Thermal Maximum (PETM), which presumably had the highest natural carbon release rate (~1.1 Pg C yr ) during the past 66 Myr on Earth (Zeebe et al., 2016). Excessive anthropogenic CO2 production and emission, mainly due to fossil fuel combustion, deforestation, and cement production, has been identified as the main cause of global climate change (Bijma et al., 2013). There have been a huge number of studies of CO2-related climate change and its environmental impacts. However, in addition to CO2, other greenhouse gases such as methane (CH4) and earth.scichina.com link.springer.com nitrous oxide (N2O) also contribute to global warming (Dickinson and Cicerone, 1986; Ravishankara et al., 2009; Dlugokencky et al., 2011; Montzka et al., 2011; Carpenter et al., 2012). Emissions of CH4 and N?O have also increased rapidly during the last few centuries, mainly due to anthropogenic activities (Forster et al., 2007; Bakker et al., 2014). Although it may be possible to keep anthropogenic CO2 emission under control in the future, the global warming effect of other greenhouse gases will still result in a continuous increase of the global temperature (Solomon et al., 2010; Montzka et al., 2011). To achieve a successful global change mitigation it is necessary to take all of the major greenhouse gases into consideration.
Methane is the second most potent greenhouse gas contributing ?20% to the present-day anthropogenic forcing of well mixed greenhouse gases relative to the pre-industrial era (Wuebbles and Hayhoe, 2002; Montzka et al., 2011; Kirschke et al., 2013). Although the atmospheric CH4 concentration (~1.83 ppm) is much lower than that of CO2 (?400 ppm), the anthropogenic CH4 radiative forcing is about 1/4 to 1/3 that of anthropogenic CO2 (Etminan et al., 2016; Ruppel and Kessler, 2017). Due to excess anthropogenic emissions, the atmospheric CH4 concentration is increasing at ?2% per year (Rasmussen and Khalil, 1981). The current atmospheric CH4 concentration is the highest over at least the past0.8 Ma and has increased2.5 times since the Industrial Revolution, which is mainly attributable to anthropogenic activities (Wuebbles and Hayhoe, 2002; Loulergue et al., 2008; Dlugokencky et al., 2011). In comparison, the atmospheric CO2 concentration has increased by less than 50% during the same period. Therefore, it has been reported that CH4 plays a prominent role in global warming (Ruppel and Kessler, 2017). This viewpoint is further substantiated by the positive feedback loop between CH4 and global warming (MacDougall and Knutti, 2016). The estimation of the global CH4 emission is about 500-600 Tg yr 1 (Conrad, 2009; Ghosh et al., 2015; Tsuruta et al., 2017). Under the impact of global warming, CH4 emissions from both natural and anthropogenic sources are expected to increase (Montzka et al., 2011; Hamdan and Wickland, 2016), which in turn will exert an even more intense influence on the rise in global temperature.
Methane also plays a very important role in modulating atmospheric chemistry. In the troposphere, CH4 is oxidized by the hydroxyl radical (OH?)and this process leads to ozone pollution (Montzka et al., 2011; Prather and Holmes, 2017). Methane oxidation contributes to the buildup of stratospheric water vapor (H2O), which is also a greenhouse gas causing global warming (Isaksen et al., 2011). In addition to creating a sink for atmospheric CH4, OH- has a major role in the destruction of some other tropospheric greenhouse gases, such as hydrofluorocarbons and hydrochlorofluorocarbons (Montzka et al., 2011). Moreover, OH- can oxidize tropospheric sulfur dioxide (SO2), forming sulfate aerosols, which have a certain cooling effect on the global climate (Montzka et al., 2011). The consumption of OH- by CH4 may thus create another positive feedback on global warming, by increasing the lifetime of atmospheric CH4 and changing the dynamics of some other tropospheric greenhouse gases (Isaksen et al., 2011; Montzka et al., 2011). Moreover, CH4 also contributes to the increase in the atmospheric CO2 concentration, with CO2 being the end product of CH4 oxidation (Isaksen et al., 2011). Furthermore, CH4 may exert an important radiative forcing-induced long-term (>200 years) effect on the ongoing sea-level rise through thermal expansion (Zickfeld et al., 2017), even if its anthropogenic emission can be halted and even though its atmospheric lifetime is only about 9 years (Sonnemann and Grygalashvyly, 2014). Overall, CH4 may play an increasingly more important role in the Earth's climate and environment systems in the future.
The global warming potential (GWP) of CH4 over a 100year period is over 20 times stronger than that of CO2. However, to keep global warming under control in the near future (i.e., a couple of decades), the GWP of CH4 over a 20- year period is more relevant to consider and is over 70 times stronger than that of CO2 (Wuebbles and Hayhoe, 2002; Karthikeyan et al., 2015). Although the task of finding a solution in this short time is tremendously challenging and difficult, there is a need to act quickly and early to avoid the danger incurred by global change and to not step over the threshold and lose the opportunity (Hansen et al., 2007; MacCracken, 2008). It has been proposed that the current anthropogenic activity-induced global warming may be irreversible and there are serious concerns that a domino effect may be triggered, causing the Earth to spiral out of balance and generating uninhabitable conditions, if we cannot act quickly and decisively to stop climate change in the near future (e.g., Frondel et al., 2002; Solomon et al., 2009; Hansen et al., 2013; Schleuning et al., 2016). Because the steady-state lifetime of CH4 in the atmosphere is much shorter than that of CO2, the reduction of CH4 emissions (and other greenhouse gases with short lifetimes) should effectively and quickly help mitigate climate change (Montzka et al., 2011; Karol' et al., 2013). The reduction in radiative forcing through the removal of atmospheric CH4 and other short-lived greenhouse gases is much faster than that of CO2 (Zickfeld et al., 2017). Although a diverse range of natural and anthropogenic sources of CH4 emissions have been identified (Figure 1), the mechanisms and contributions of some of the sources to atmospheric CH4 have not been clearly resolved, and this is particularly true for the ocean (Kirschke et al., 2013; Hamdan and Wickland, 2016). This situation hinders a mechanistic and comprehensive prediction of the global warming caused by CH4 and its application in climate change mitigation.
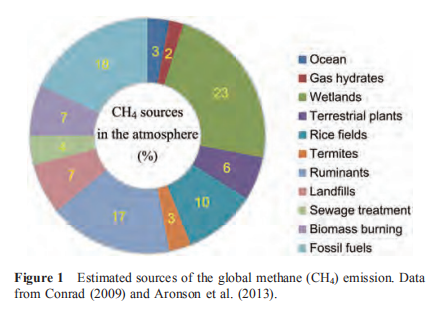
2.The ocean as a net source of to the atmosphere
In general, the ocean contributes to net sequestration of anthropogenic CO?. It has been estimated that ?40% of the anthropogenic CO? emissions since the Industrial Revolution have been absorbed by the ocean (Sabine et al., 2004; McKinley et al., 2016; DeVries et al., 2017). However, the ocean is generally regarded to be a minor contributor to atmospheric CH4, based on various estimations (Bange et al., 1994; Matthews, 1994; Kruger et al., 2005; Carpenter et al., 2012). The ocean-to-atmosphere emission rate of CH4 is estimated to be ?1.3 to 13 Tg yr 1 (Ruppel and Kessler, 2017). However, the wide range of estimations indicates that these estimations contain much uncertainty due to a lack of sufficient sampling coverage and long time-series measurements of the global ocean, and they are unable to capture the temporospatial dynamics of CH4 emissions in the ocean (Bange, 2006; Fischer et al., 2013; Hamdan and Wickland,2016). The sea-to-air CH4 flux varies substantially among different marine environments and the mechanisms that control the huge temporal and spatial variation of CH4 emissions are still not fully resolved (Bates et al., 1996; Ortiz-Llorente and Alvarez-Cobelas, 2012; Wilson et al.,2017). The lack of long-term measurements with sufficient temporal and spatial frequencies and resolutions, in particular at regional and global scales, hinders global marine CH4 data integration, and thus an accurate estimation of marine CH4 emissions from the global ocean.
Compared to the ocean, the global wetlands are a much larger source of CH4 emissions (Bridgham et al., 2013). A recent study reported that the average CH4 emission from wetlands on a global scale was 177.2 49.7 Tg CH4 yr for the period from 2000 to 2007 (Zhang et al., 2017). As the land-ocean interface, coastal wetlands also exert a strong influence on CH4 emissions from coastal marine waters. Due to the large terrestrial, fluvial, and anthropogenic inputs of organic matter and nutrients, intense microbial activities,including methanogenesis, occur in coastal wetlands (Vizza et al., 2017; Xiao L et al., 2017). Although coastal wetlands, defined as areas of marine water with a water depth not exceeding 6 m at low tide (Navid, 1989), account for only a small fraction of the global ocean system, their CH4 production may constitute a major source of CH4 emissions in adjacent estuarine and coastal seas (Wang et al., 2016).
In the ocean, many estuarine and coastal waters are hotspots of CH4 emission. This is usually caused by intense terrestrial and sediment inputs and in situ microbial methanogenesis under hypoxic and anoxic conditions (Zhang et al., 2004, 2008; Bange, 2006; Zhou et al., 2009; Borges et al., 2016; Upstill-Goddard and Barnes, 2016; Farias et al., 2017; Sela-Adler et al., 2017; Tseng et al., 2017). In addition to the direct transport of terrigenous CH4, river discharges usually contain high concentrations of organic matter and nutrients. This can stimulate various forms of biological production and respiration in the estuarine and coastal microbial communities and create locally and/or seasonally hypoxic and even anoxic conditions (Naqvi et al., 2010; Dang and Jiao, 2014). Estuarine and coastal seawater commonly contains high concentrations of suspended particles, likely harboring hypoxic and anoxic microenvironments inside the particles for anaerobic microbial processes (Brooks et al., 1981; Wright et al., 2012; Dang and Jiao, 2014; Dang and Lovell, 2016). The oxygen-depleted environments and microenvironments in seawater facilitates CH4 production by anaerobic archaeal methanogens and sea-to-air CH4 emission (Offre et al., 2013; Wen et al., 2017). Although the estuarine and coastal seas only occupy a small fraction of the global ocean, they may contribute ?75% of the total marine CH4 emissions to the atmosphere (Bange et al., 1994; Reeburgh, 2007).
Coastal upwelling can bring CH4 from the bottom water into surface water and contributes directly to CH4 emission (Bange etal., 1998; Capelle and Tortell, 2016; Chronopoulou et al., 2017). In addition, the enrichment of nutrients by upwelling stimulates the biological production of organic matter and microbial community respiration, creating hypoxic and anoxic environments in seawater for anaerobic methanogenesis (Sansone et al., 2001; Wright et al., 2012; Bakun, 2017; Shepherd et al., 2017). Therefore, the hypoxic and anoxic zones of the marginal sea upwelling areas are environments of variable CH4 production and emission. Climate change may intensify coastal upwelling, particularly at high latitudes (Sydeman et al., 2014; Wang et al., 2015). This change may exert a strong impact on the structures and functions of the coastal marine ecosystems, including the intensified production and emission of greenhouse gases such as CH4 and N2O.
Sediments are a major source of seawater CH4 (Orcutt et al., 2013; Chronopoulou et al., 2017; Tseng et al., 2017; Xiao K Q et al., 2017). The anoxic conditions in bulk sedimentsfacilitate anaerobic microbial metabolisms, including methanogenesis (Ferry and Lessner, 2008; Sela-Adler et al., 2017). Sediments in marginal seas receive high inputs of organic matter that contribute to microbiogenic CH4 production (Wen et al., 2017). Many marginal sea sedimentary environments in the world's oceans harbor methane hydrates (also called gas hydrates). Because they are metastable and have a high sensitivity to warming, gas hydrates are a net source of CH4 emission to the atmosphere (Conrad, 2009). Although the estimated contribution of CH4 emission (~6 Tg yr 1, ranging from 2 to 9 Tg yr 1) from marine gas hydrates to atmospheric CH4 is currently small (Ruppel and Kessler, 2017), gas hydrates have the potential to release a large amount of CH4 in a relatively short period of time, and thus they may constitute a tipping point in future global climate change (Archer et al., 2009; Carpenter et al., 2012).
3.Gas hydrate CH4 emission as a looming factor influencing climate change
In certain sediments, such as those harboring gas hydrates, the CH4 seepage can be phenomenal (Boetius and Wenzho- fer, 2013; Rakowski et al., 2015). For example, CH4 gas bubbles rising through seawater can reach 400 m above the seafloor where active seepage sites exist (Dang et al., 2010). Both biogenic and thermogenic CH4-producing processes can contribute to the accumulation of CH4 in marine sediments (Archer, 2007; Reeburgh, 2007; Hester and Brewer, 2009). With a favorable gas composition and under low- temperature and high-pressure conditions, methane hydrates can form in marine sediments (Ruppel and Kessler, 2017). It has been estimated that marine gas hydrates may contain a huge amount of CH4, ranging from 1700 to 4100000 Gt C globally (Kvenvolden, 1988). The latest estimations suggest that the inventory of marine methane hydrates may be at the lower end of the original estimates, about 1100-2000 Gt C (Archer et al., 2009; Boswell and Collett, 2011; Kretschmer et al., 2015; Ruppel and Kessler, 2017). Because the spatial distribution of sediment gas hydrates is extremely heterogeneous in the ocean and there is generally a lack of sampling coverage across the global ocean, it is not easy to make an accurate estimate of gas hydrate resources (Marin-Moreno et al., 2016), nor is it easy to estimate the CH4 emission fluxes from the globally dispersed gas hydrate sources (Matthews, 1994).
Although there may be a huge amount of CH4 dissociated from the seafloor gas hydrates every year, a variable (and usually small) fraction of hydrate CH4 may actually enter the atmosphere. It is commonly estimated that hydrate CH4 may contribute only ?2% to the pool of atmospheric CH4 (Matthews, 1994; Conrad, 2009; Hamdan and Wickland, 2016; Ruppel and Kessler, 2017). Anaerobic and aerobic metha- notrophic microbes (including bacteria and archaea) consume the majority of the free CH4 pool in sediments and seawater, presumably substantially lowering the global sea- to-air CH4 flux (Kruger et al., 2005; Reeburgh, 2007; Knittel and Boetius, 2009; DiSpirito et al., 2016; James et al., 2016). To facilitate CH4 metabolism, many microbial methano- trophs harbor N2-fixation genetic inventories to provide biologically fixed nitrogen (Auman et al., 2001; Dedysh et al., 2004; Pernthaler et al., 2008; Dang et al., 2009; Dekas et al., 2009; Khadem et al., 2010; Fernandez-Carrera et al.,
2016). This genetic and biochemical adaptation strategy indicates that marine methanotrophic microbes may have evolved in CH4-rich marine environments throughout the Earth's history.
Recent studies have identified a highly variable contribution of hydrate CH4 emissions to global warming (Biastoch et al., 2011; Kretschmer et al., 2015; Mestdagh et al., 2017). The massive CH4 emissions putatively caused by abrupt seafloor gas hydrate dissociation are considered to be a very important factor contributing to global warming during certain historical events in the Earth's history, including the PETM and the termination of the Marinoan “Snowball" ice age (Dickens et al., 1995, 1997; Kennett et al., 2003; Maslin et al., 2004; Svensen et al., 2004; Kennedy et al., 2008; Dickens, 2011). Catastrophic CH4 emissions associated with massive and abrupt marine gas hydrate dissociation are also hypothesized to have been involved in mass extinctions (Katz et al., 1999; Norris and Rohl, 1999; Hesselbo et al., 2000). Although the “clathrate gun hypothesis" has been challenged (Higgins and Schrag, 2006; Sowers, 2006; Gut- jahr et al., 2017), CH4 emission from dissociated gas hydrates caused by gradually warming seawater over timescales of tens of thousands of years may still have an important impact on the climate (Archer et al., 2009). Leifer et al. (2006) identified natural marine seepage blowout as a process enabling rapid climate change by sudden hydrate dissociation-related massive methane bubble releases. Furthermore, the oxidation of CH4 released from dissociated gas hydrates consumes O2 and produces CO2, leading to ocean deoxygenation and acidification (Biastoch et al., 2011; Boudreau et al., 2015).
The metastable nature of gas hydrates indicates that they are sensitive to changes in temperature and pressure, while sediment gas hydrates can be dissociated by environmental disturbances, such as a rising seawater temperature (Reagan and Moridis, 2007; Hunter et al., 2013; Ruppel and Kessler,2017). It has been hypothesized that CH4 emission from gas hydrate dissociation may become stronger with an increase in the global and ocean temperature (Kvenvolden, 1988). There is evidence that the ocean's interior is getting warmer due to the impact of global warming (Masuda et al., 2010; Mora et al., 2013; Levin and Le Bris, 2015). Modeling studies have indicated that CH4 emissions from marine gas hydrates are increasing under the influence ofongoing global warming, particularly in certain sensitive environments such as the Arctic Ocean and shallow marginal seas (Reagan and Moridis, 2007; Shakhova et al., 2010; Isaksen et al., 2011; Marin-Moreno et al., 2013; Thatcher et al., 2013; James et al., 2016). There have been observations and model predictions that CH4 is released from gas hydrates to the atmosphere in response to global warming (Archer, 2007; Thatcher et al., 2013; Stranne et al., 2017). Global change is not a simple process, and actually involves nonlinear and sometimes abrupt changes of the Earth system and its components (McNeall et al., 2011). This complexity suggests that a small change in a particular external forcing could cause large and/or irreversible changes to climate (McNeall et al., 2011). For example, methane seepages from seafloor sediments are frequently and widely found to be episodic in many different marine environments (Stranne et al., 2017). In addition to the effects caused by the rising bottom water temperature due to global warming, there are other processes and factors in the ocean that may influence the stability and CH4 emission of sediment gas hydrates. The current climate change may eventually alter the ocean circulation pattern and the intensity of some currents. For example, changes in the Gulf Stream intermediate water temperature could cause a rapid destabilization of gas hydrates along a broad path in the North American margin seas (Phrampus and Hornbach, 2012). Submarine volcano eruptions may release CH4 from gas hydrates if these two geological features happen to exist in the same area (Svensen et al., 2004). This is highly likely because most (?80%) of the Earth's volcanic activity happens in submarine environments (Embley et al., 2006). Large-scale submarine landslides are a major geohazard that can trigger basin-wide tsunamis. Earthquakes, volcanoes, hurricanes, and gas hydrate dissociation can trigger submarine slides (Masson et al., 2006; Geissler et al., 2016; Handwerger et al., 2017), which in turn may cause further large-scale gas hydrate dissociation and CH4 releases. Earthquakes can also directly cause seafloor ruptures, gas hydrate dissociation, and an intensification of methane seepage (Tsunogai et al., 2012; Fischer et al., 2013; Obzhirov, 2013; Geersen et al., 2016). Global warming may impact on some of these submarine geohazard processes. For example, the warming of bottom seawater may destabilize gas hydrates, which in turn may lead to submarine landslides and lead to further hydrate CH4 emissions. This scenario may occur in the Arctic Ocean and some other shallow seas. In Greenland and the Antarctic, another mechanism may operate. Here, global warming has caused the shrinking of ice sheets and a rise in the affected continental slope through isostacy. The lowered hydrostatic pressure may destabilize gas hydrates, leading to massive landslides and gas hydrate dissociation (Maslin et al., 2010). In addition, the commercial exploitation of offshore oils and gas hydrates may also trigger gas hydrate dissociation and CH4 release (Glasby, 2003; Zhang and Zhai, 2015; Fernandez-Carrera et al., 2016). The positive feedback effect of hydrate CH4 emission on global warming and the various processes that could trigger large-scale “catastrophic" CH4 releases from gas hydrates has led to serious concerns about the impact of gas hydrates on the global climate (Reagan and Moridis, 2008; Archer et al., 2009; Carpenter et al., 2012; Ruppel and Kessler, 2017).
4.The ocean methane paradox and putative mechanisms
Many microorganisms produce greenhouse gases that exacerbate global warming. For example, it has been estimated that methanogenic archaea contribute nearly 60% of the methane emissions that are related to anthropogenic activities (Jablonski et al., 2015). Methane was once believed to be produced exclusively by methanogenic archaea affiliated with the phylum Euryarchaeota (i.e., the Methanobacteria, Methanococci, Methanomicrobia, and Methanopyri classes) (Balch et al., 1979; Garcia et al., 2000; Liu and Whitman, 2008). Recently, novel euryarchaeotal methanogens, such as those affiliated with the newly defined family Candidatus Methanoflorentaceae (within class Methanomicrobia), orders Methanocellales (within class Methanomicrobia) and Methanomassiliicoccales (within class Thermoplasmata), and classes Ca. Methanofastidiosa and Methanona- tronarchaeia have been discovered (Sakai et al., 2008; Iino et al., 2013; Mondav et al., 2014; Nobu et al., 2016; Sorokin et al., 2017). In addition, non-euryarchaeotal methanogens, such as those affiliated with the new archaeal phyla Bath- yarchaeota and Verstraetearchaeota, have also been discovered recently (Evans et al., 2015; Vanwonterghem et al., 2016).
Four distinct biochemical pathways for the production of CH4 in archaeal methanogens have been identified: (1) hy- drogenotrophic methanogenesis using 玦 as an electron donor to reduce CO2 for CH4 production, (2) acetoclastic methanogenesis using acetate as the substrate for CH4 disproportionation production, (3) methylotrophic methanogenesis using methylated C1 compounds (e.g., methanol, methylamines, and methylthiols) as substrates for CH4 disproportionation production, and (4) methyl-reducing methanogenesis using methylated C1 compounds as substrates and H2 and/or formate as electron donors for CH4 production by methyl group reduction (Kallistova et al., 2017). Coenzyme M (CoM) and coenzyme F420 are central cofactors in methanogenic archaea and methyl-CoM reductase is a unique key enzymatic complex that has a function in the final step of methanogenesis (Balch et al., 1979; Friedrich, 2005; Krishnakumar et al., 2008; Purwantini et al., 2014; Dziewit et al., 2015; Greening et al., 2016). A common physiological characteristic of all the above-mentioned archaeal methanogens is that they are anaerobes, producing CH4 only in anoxic environments such as oxygen-depleted marine sediments and seawater (Valentine, 2011; Offre et al., 2013; Welte and Deppenmeier, 2014). The energy yield by traditional methanogenesis is very low (Schink, 1997; Schafer et al., 1999). In environments with the presence of other oxidants and oxidative metabolic processes, methanogens are rarely successful competitors (Sela-Adler et al., 2017; Wen et al., 2017). Surprisingly, however, most oxic surface or nearsurface waters in the world's oceans are supersaturated with CH4 relative to atmospheric CH4 concentrations. This puzzling phenomenon is referred to as the “ocean methane paradox" (Reeburgh, 2007; Karl et al., 2008). There is much evidence indicating that CH4 in these waters may be produced in situ and constitutes a net flux to the atmosphere (Lamontagne et al., 1971; Scranton and Brewer, 1977). It has been estimated that the world's open ocean CH4 emission flux to the atmosphere may be ~3.6 Tg yr 1 (Lambert and Schmidt, 1993; Bange et al., 1994), while some other studies have shown that the actual flux (~0.4 Tg yr 1) may be an order of magnitude less (Bates et al., 1996; Rhee et al., 2009). Although the estimated contribution of the open oceans to atmospheric CH4 is highly variable among different studies, it is widely accepted that the open oceans generally constitute a net CH4 source to the atmosphere, although the underlying CH4 production mechanism remains unclear and hotly debated (Reeburgh, 2007; Karl et al., 2008; Conrad, 2009; Rakowski et al., 2015; Tseng et al., 2017).
Anoxic microenvironments associated with marine particles and zooplankton guts may host active methanogenic archaea, contributing to in situ CH4 production and accumulation in oxic bulk seawater (Oremland, 1979; Brooks et al., 1981; Cynar and Yayanos, 1991; de Angelis and Lee, 1994; Karl and Tilbrook, 1994; Tilbrook and Karl, 1995; Marty et al., 1997; Holmes et al., 2000; Reeburgh, 2007; Sasakawa et al., 2008; Ditchfield et al., 2012; Dang and Lovell, 2016). Some methanogens may have developed ecophysiological adaptations to various adverse environments. For example, some methanogens can survive oxic conditions and resume methanogenic activities when the environment is conducive (Zehnder and Wuhrmann, 1977; Jarrell, 1985; Sieburth et al., 1993; Tholen et al., 2007; Poehlein et al., 2017). The surface waters of the ocean may experience a diel alternation of the dissolved oxygen content due to day-time phytoplankton oxygenic photosynthesis and night-time community oxygen-consuming respiration (Dang and Lovell, 2016). It may be easier to achieve hypoxic and even anoxic microenvironments in marine particles and biofilms, which would facilitate methanogenesis during the night. In addition, some environmental methanogen isolates harbor diverse adhesin-like proteins, which are useful for colonizing marine particles and other substrata (e.g., zooplankton surfaces and/or digestive tracts) (Dang and Lovell, 2016; Poehlein et al., 2017). Recently a novel methanogen, Candidatus Methanothrix paradoxum, was identified in oxygenated soils, and it was shown to conduct acetoclastic CH4 production in the anoxic microenvironments of soil aggregates (Angle et al., 2017). The novel feature of this archaeon is its ability to compete for substrate acquisition under low acetate conditions, which provides an ecophy- siological advantage over other methanogens in bulk oxic environments. Archaeal 16S rRNA gene sequences that are very similar to that of Ca. Methanothrix paradoxum are prevalent in oxic permafrost, freshwater, wetland, estuary, and marine environments (Angle et al., 2017), and some sequences were obtained from the prokaryotic communities associated with marine snow (Vojvoda et al., 2014). The discovery of this specific methanogen indicates that the anoxic microenvironment hypothesis is likely to be valid for explaining the “ocean methane paradox", at least for certain marine waters.
Methane production also occurs in particle-free oxygenated seawater (Bogard et al., 2014), and certain studies have found that zooplankton contribute very little to oxic CH4 production (Schmale et al., 2018). The ocean methane paradox was further tentatively resolved by the finding that some marine bacteria, including cyanobacteria, can metabolize methylphosphonate (MPn), producing CH4 as a byproduct for phosphorus acquisition (Karl et al., 2008; Dyhrman et al., 2009; Beversdorf et al., 2010; Martinez et al., 2013; Carini et al., 2014; del Valle and Karl, 2014; Re- peta et al., 2016; Horsman and Zechel, 2017; Sosa et al., 2017; Teikari et al., 2018). In addition, it has been shown that marine snow particles are hotspots for MPn degradation and CH4 production (del Valle and Karl, 2014). MPn is produced abundantly by thaumarchaeotal ammonia-oxidizing archaea (AOA), cyanobacteria and some other marine microorganisms (Dyhrman et al., 2009; Metcalf et al., 2012; Van Mooy et al., 2015; Dang and Chen, 2017). In addition to bacteria, some archaea have also been found to harbor the gene encoding the carbon-phosphorus lyase, which is responsible for CH4 production from substrates such as MPn (Hove-Jensen et al., 2014). The team work performed by different microorganisms during MPn production and consumption (Dang et al., 2013), particularly in phosphate-starved environments, may produce a large pool of surface seawater CH4 in the global ocean and contribute substantially to the net sea-to-air CH4 flux.
Although CH4 production associated with MPn and particle microenvironments is intriguing and likely important, some alternative microbial processes have also been proposed to account for CH4 production in oxygenated seawater. Methane may also be produced during the microbial meta- bolization of dimethylsulfoniopropionate (DMSP) and its intermediate degradation products, such as dimethylsulfide (DMS), methanethiol (MeSH), and methylmercaptopropionate (MMPA) (Welsh, 2000; Damm et al., 2008, 2010; Florez-Leiva et al., 2013; Weller et al., 2013; Zindler et al., 2013). Certain methanogens synthesize methylthiol:CoM methyltransferase or other substrate-specific CoM methyltransferases, using methylated sulfur compounds (MSCs, e.g., DMS, MeSH, and MMPA) as substrates for CH4 production (Tallant and Krzycki, 1997; Tallant et al., 2001; Fu and Metcalf, 2015). Many methanogens have been isolated and shown to grow with MSCs for methylotrophic methanogenesis (Kiene et al., 1986; Oremland et al., 1989; Finster et al., 1992; van der Maarel and Hansen, 1997; Lomans et al., 1999; Lyimo et al., 2000; Cha et al., 2013; Fu and Metcalf,2015). However, all these archaeal strains produce CH4 only under anoxic conditions. Whether and how the MSC-utiliz- ing anaerobic methylotrophic methanogens can cope with oxygen stress under oxic conditions in the surface waters of the ocean is currently unresolved. A recent comparative genomic analysis revealed differences in oxygen tolerance among methanogenic archaea, suggesting that the Class II methanogens may be more adapted to microaerophilic environments than Class I methanogens (Lyu and Lu, 2018). In addition, it has been proposed that certain aerobic bacteria may use DSMP as a carbon source and produce CH4 as a byproduct in oxic marine waters (Damm et al., 2010). The MSC-related CH4-producing mechanisms may constitute an important pathway in the ocean-to-atmosphere CH4 flux and in marine C and S cycling. DMSP is an abundant phytoplankton and coral metabolite that plays an important role in protecting organisms from osmotic, oxidative, and thermal stresses (Bullock et al., 2017). Methane is a potent greenhouse gas (Bogard et al., 2014), while DMS is the most important biogenic climate-cooling gas in nature (Carini,2016). Phytoplankton blooms are a global environmental and ecological problem in the coastal oceans of the world (Dang and Jiao, 2014; Dang and Lovell, 2016; Dang and Chen,2017). With increasing anthropogenic impacts, such as excess nutrient discharges into the ocean, phytoplankton blooms will occur more frequently and extensively, particularly in estuarine and coastal seas (Jiao et al., 2014). More DMSP will therefore be produced by phytoplankton in the marginal seas in response to ocean eutrophication (Dang and Lovell, 2016). Under this scenario, it is reasonable to hypothesize that the conversion of DMS to CH4 by marine microorganisms may further tip the balance of the Earth's thermal budget and result in faster and stronger global warming (Florez-Leiva et al., 2013).
In addition to the three major hypotheses regarding microbial processes and mechanisms for the paradoxical production of CH4 in oxic ocean waters mentioned above, some other hypotheses have also been proposed. Terrestrial plants are a major source of CH4 (Conrad, 2009). Recently, the coccolithophore Emiliania huxleyi has been found to produce CH4 directly, without the involvement of archaeal methanogens or CH4-producing bacteria (Lenhart et al., 2016). Emiliania huxleyi is widespread and is the most abundant calcifying phytoplankton in the ocean where it plays an important role in ocean carbon sequestration (Krumhardt et al., 2017). Its contribution to CH4 production in oxic surface waters, and thus to the ocean's CH4 flux to the atmosphere requires an in-depth and systematic investigation. Damm et al. (2015) recently proposed another hypothesis about the mechanism of CH4 production in oxic nitrate-stressed marine environments. Bacterial respiration and low membrane permeability may create and maintain intracellular anaerobic conditions, which would be conducive to DMSP-dependent CH4 formation inside bacterial cells despite the oxic conditions of the surrounding bulk seawater (Damm et al., 2015). This has been further supported by a modeling analysis of single-cell metabolic processes (Damm et al., 2015).
Regarding the ocean methane paradox, it is likely that most of the microbial processes and mechanisms for CH4 production in oxic seawater proposed above may be valid. They may function under different environmental and/or biological conditions. However, it is still not quite clear what the environmental or biological determinants are that drive these differences. Currently, their respective contributions to the seawater CH4 budget and sea-to-air CH4 emission are not clear, while their quantitative effect on the marine phosphorus and sulfur cycles also remains unclear. These uncertainties highlight the challenges that remain in marine microbiota and global change research and present opportunities for future scientific breakthroughs.
5.Future perspectives on marine research
Global climate change induced by greenhouse gases isavery complex process. There are complicated interactions and positive feedback effects involved that make global warming very difficult to mitigate. Methane as a potent greenhouse gas plays an important role in raising the global temperature. However, many features of the processes and mechanisms involved in the biogeochemical cycling relating to this simple organic molecule remain unresolved. Methane emission via marine sediment gas hydrate dissociation constitutes a net source of atmospheric CH4. Recent studies have revealed certain unexpected ecological and climate impacts of CH4 released this way. It has recently been found that the upwelling produced by ascending CH4 gas bubbles moves deep-water nutrients into the surface water, and thus enhances photosynthetic CO2 fixation on the Svalbard margin (Pohlman et al., 2017). A recent study has indicated that massive emissions of CH4 from seep areas may be very common in shallow coastal seas (Borges et al., 2016). Although CH4 emissions are unavoidably large, coastal marine environments, such as the Svalbard margin and others where similar CHq-related geological and ecological processes occur, may constitute net greenhouse gas sinks, and thus have a cooling effect on the regional and global climate (Pohlman et al., 2017).
Several lessons have already been learned from history. One ofthese isthatthe real world is often more complex than was initially imagined. There are many uncertainties and unknowns regarding marigenic CH4 in the process of global warming. Despite this, our understanding of the roles played by CH4 in ocean carbon cycling and global climate change has evolved. For example, regardless of what the processes and mechanisms are behind the paradoxical CH4 production in oxic marine waters, microbial activities always seem to be involved (Reeburgh, 2007). The diversity of microbes, their metabolic pathways, and environmental adaptation strategies results in complexity among the processes and functions of natural ecosystems, including CH4 production in oxic marine environments.
Under the influence of ongoing global and marine environmental changes, certain microbial processes may be altered. Global warming may have a direct impact on global microbial methanogenesis, which has been found to be more sensitive and responsive to a change in temperature than respiration and photosynthesis (Yvon-Durocher et al., 2014). With the projected rise in ocean temperature, it has been suggested that the increase in marine CH4 production and emission will be stronger than that ofCO2 (Yvon-Durocher et al., 2014). Increased anthropogenic activities and ocean warming will exacerbate the hypoxic conditions in most estuarine and coastal seas and in oceanic oxygen minimum zones (Gruber, 2011; Gilly et al., 2013; Dang and Jiao, 2014; Dang and Lovell, 2016; Dang and Chen, 2017). Some of the hypoxic waters may develop anoxic cores that will facilitate microbial CH4 production. Under the influence of global climate change, upwelling in certain areas of the ocean will be enhanced (Sydeman et al., 2014; Wang et al., 2015). This may create hypoxic and even anoxic water bodies that facilitate methanogenesis (Bakun, 2017). Strengthened upwelling also stimulates the formation of phytoplankton blooms (Kudela et al., 2010), enhancing CH4 production via the enhanced production of DMSP and increased formation of marine particles (Damm et al., 2008, 2010; Florez-Leiva et al., 2013; Weller et al., 2013; Dang and Lovell, 2016). Moreover, increased ocean acidification may reduce nitrification in the ocean, because more environmental ammonia would be converted to ammonium, which would reduce the ammonia oxidation rate by AOA and ammoniaoxidizing bacteria (AOB) in both seawater and sediments (Beman et al., 2011; Braeckman et al., 2014; Dang and Chen, 2017). How this may influence the production of MPn by AOA (but not by AOB) and the related production of CH4 is currently unknown. Furthermore, methanogenic and metha- notrophic microbes control the production and consumption of CH4, and thus its abundance and dynamics in marine environments. Certain trace elements (e.g., Co, Cu, Fe, and Ni) are important cofactors for enzymes involved in microbial methanogenesis and methanotrophy (Glass and Orphan, 2012; DiSpirito et al., 2016; Paulo et al., 2017; Semrau et al., 2018). The bioavailability of these trace elements in the ocean may play an important role in the microbial activities involved in CH4 production and consumption. On the other hand, heavy metals may exert negative impacts on the activities of microbial methanogens and methanotrophs via enzyme toxification. Ocean warming, acidification, deoxygenation, eutrophication, and pollution may all influence the availability of trace elements, and the effects of heavy metals in marine environments (Dang and Chen, 2017). The association of microbial activity with marine inorganic elements and contaminants makes the prediction of changes in the future climate and marine environment even more complex and difficult. The advancement of “omics" techniques, such as metagenomics, metatranscriptomics, and metaproteomics, provides opportunities for the study and understanding of the microbes and their processes and mechanisms in the ocean's CH4 dynamics (Lloyd, 2015). The combination of the “omics" analyses with in situ flux measurements may enable the development of more powerful and accurate biogeochemical models, resulting in a better prediction of the ocean's CH4 behavior and its influence on global change.
Acknowledgements This work was supported by the National Key Research and Development Program of China (Grant No. 2016YFA0601303), the Chinese State Oceanic Administration (SOA) (Grant No. GASI-03-01-02-05), the National Natural Science Foundation of China (Grant Nos. 41676122, 91328209 & 91428308), and the China National Offshore Oil Corporation (Grant Nos. CNOOC-KJ125FZDXM00TJ001- 2014 & CNOOC-KJ125FZDXM00ZJ001-2014).
References
Angle J C, Morin T H, Solden L M, Narrowe A B, Smith G J, Borton M A, Rey-Sanchez C, Daly R A, Mirfenderesgi G, Hoyt D W, Riley W J, Miller C S, Bohrer G, Wrighton K C. 2017. Methanogenesis in oxygenated soils is a substantial fraction of wetlandmethane emissions. Nat Commun, 8: 1567
Archer D. 2007. Methane hydrate stability and anthropogenic climate change. Biogeosciences, 4: 521-544
Archer D, Buffett B, Brovkin V. 2009. Ocean methane hydrates as a slow tipping point in the global carbon cycle. Proc Natl Acad Sci USA, 106: 20596-20601
Aronson E L, Allison S D, Helliker B R. 2013. Environmental impacts on the diversity of methane-cycling microbes and their resultant function. Front Microbiol, 4: 225
Auman A J, Speake C C, Lidstrom M E. 2001. nifH sequences and nitrogen fixation in type I and type II methanotrophs. Appl Environ Microbiol, 67: 4009-4016
Bakker D C E, Bange H W, Gruber N, Johannessen T, Upstill-Goddard R C, Borges A V, Delille B, Loscher C L, Naqvi SWA, Omar A M, Santana-Casiano J M. 2014. Air-sea interactions of natural long-lived greenhouse gases (CO2, N?。,CH4) in a changing climate. In: Liss P S, Johnson M T, eds. Ocean-Atmosphere Interactions of Gases and Particles. Heidelberg: Springer
Bakun A. 2017. Climate change and ocean deoxygenation within intensified surface-driven upwelling circulations. Phil Trans R Soc A, 375: 20160327
Balch W E, Fox G E, Magrum L J, Woese C R, Wolfe R S. 1979. Methanogens: Reevaluation of a unique biological group. Microbiol Rev, 43: 260-296
Bange H W. 2006. Nitrous oxide and methane in European coastal waters. Estuar Coast Shelf Sci, 70: 361-374
Bange H W, Bartell U H, Rapsomanikis S, Andreae M O. 1994. Methane in the Baltic and North Seas and a reassessment of the marine emissions of methane. Glob Biogeochem Cycle, 8: 465-480
Bange H W, Ramesh R, Rapsomanikis S, Andreae M O. 1998. Methane in surface waters of the Arabian Sea. Geophys Res Lett, 25: 3547-3550
Bates T S, Kelly K C, Johnson J E, Gammon R H. 1996. A reevaluation of the open ocean source of methane to the atmosphere. J Geophys Res, 101: 6953-6961
Beerling D J, Royer D L. 2011. Convergent Cenozoic CO? history. Nat Geosci, 4: 418-420
Beman J M, Chow C E, King A L, Feng Y, Fuhrman J A, Andersson A, Bates N R, Popp B N, Hutchins D A. 2011. Global declines in oceanic nitrification rates as a consequence of ocean acidification. Proc Natl Acad Sci USA, 108: 208-213
Beversdorf L J, White A E, Bjorkman K M, Letelier R M, Karl D M. 2010. Phosphonate metabolism by Trichodesmium IMS101 and the production of greenhouse gases. Limnol Oceanogr, 55: 1768-1778
Biastoch A, Treude T, Rupke L H, Riebesell U, Roth C, Burwicz E B, Park W, Latif M, Boning C W, Madec G, Wallmann K. 2011. Rising Arctic Ocean temperatures cause gas hydrate destabilization and ocean acidification. Geophys Res Lett, 38: L08602
Bijma J, Portner H O, Yesson C, Rogers A D. 2013. Corrigendum to “Climate change and the oceans—What does the future hold?" Mar Pollut Bull, 76: 436
Boetius A, Wenzhofer F. 2013. Seafloor oxygen consumption fuelled by methane from cold seeps. Nat Geosci, 6: 725-734
Bogard M J, del Giorgio P A, Boutet L, Chaves M C G, Prairie Y T, Merante A, Derry A M. 2014. Oxic water column methanogenesis as a major component of aquatic CH4 fluxes. Nat Commun, 5: 5350
Borges AV, Champenois W, Gypens N, Delille B, Harlay J. 2016. Massive marine methane emissions from near-shore shallow coastal areas. Sci Rep, 6: 27908
Boswell R, Collett T S. 2011. Current perspectives on gas hydrate resources. Energy Environ Sci, 4: 1206-1215
Boudreau B P, Luo Y, Meysman F J R, Middelburg J J, Dickens G R. 2015. Gas hydrate dissociation prolongs acidification of the Anthropocene oceans. Geophys Res Lett, 42: 9337-9344A
Braeckman U, Van Colen C, Guilini K, Van Gansbeke D, Soetaert K, Vincx M, Vanaverbeke J. 2014. Empirical evidence reveals seasonally dependent reduction in nitrification in coastal sediments subjected to near future ocean acidification. Plos One, 9: e108153
Bridgham S D, Cadillo-Quiroz H, Keller J K, Zhuang Q. 2013. Methane emissions from wetlands: Biogeochemical, microbial, and modeling perspectives from local to global scales. Glob Change Biol, 19: 13251346
Brigham-Grette J, Melles M, Minyuk P, Andreev A, Tarasov P, DeConto R, Koenig S, Nowaczyk N, Wennrich V, Rosen P, Haltia E, Cook T, Gebhardt C, Meyer-Jacob C, Snyder J, Herzschuh U. 2013. Pliocene warmth, polar amplification, and stepped Pleistocene cooling recorded in NE Arctic Russia. Science, 340: 1421-1427
Brooks J M, Reid D F, Bernard B B. 1981. Methane in the upper water column of the northwestern Gulf of Mexico. J Geophys Res, 86: 1102911040
Bullock H A, Luo H, Whitman W B. 2017. Evolution of dimethylsulfo- niopropionate metabolism in marine phytoplankton and bacteria. Front Microbiol, 8: 637
Capelle D W, Tortell P D. 2016. Factors controlling methane and nitrous- oxide variability in the southern British Columbia coastal upwelling system. Mar Chem, 179: 56-67
Carini P. 2016. Microbial oxidation of DMS to DMSO: A biochemical surprise with geochemical implications. Environ Microbiol, 18: 23022304
Carini P, White A E, Campbell E O, Giovannoni S J. 2014. Methane production by phosphate-starved SAR11 chemoheterotrophic marine bacteria. Nat Commun, 5: 4346
Carpenter L J, Archer S D, Beale R. 2012. Ocean-atmosphere trace gas exchange. Chem Soc Rev, 41: 6473-6506
Cha I T, Min U G, Kim S J, Yim K J, Roh S W, Rhee S K. 2013. Methanomethylovorans uponensis sp. nov., a methylotrophic methanogen isolated from wetland sediment. Antonie van Leeuwenhoek, 104: 1005-1012
Chronopoulou P M, Shelley F, Pritchard W J, Maanoja S T, Trimmer M. 2017. Origin and fate of methane in the Eastern Tropical North Pacific oxygen minimum zone. ISME J, 11: 1386-1399
Conrad R. 2009. The global methane cycle: Recent advances in understanding the microbial processes involved. Environ Microbiol Rep, 1: 285-292
Cynar F J, Yayanos A A. 1991. Enrichment and characterization of a methanogenic bacterium from the oxic upper layer of the ocean. Curr Microbiol, 23: 89-96
Damm E, Helmke E, Thoms S, Schauer U, Nothig E, Bakker K, Kiene R P. 2010. Methane production in aerobic oligotrophic surface water in the central Arctic Ocean. Biogeosciences, 7: 1099-1108
Damm E, Kiene R P, Schwarz J, Falck E, Dieckmann G. 2008. Methane cycling in Arctic shelf water and its relationship with phytoplankton biomass and DMSP. Mar Chem, 109: 45-59
Damm E, Thoms S, Beszczynska-Moller A, Nothig E M, Kattner G. 2015. Methane excess production in oxygen-rich polar water and a model of cellular conditions for this paradox. Polar Sci, 9: 327-334
Dang H Y, Chen C T A. 2017. Ecological energetic perspectives on responses of nitrogen-transforming chemolithoautotrophic microbiota to changes in the marine environment. Front Microbiol, 8: 1246
Dang H Y, Lovell C R. 2016. Microbial surface colonization and biofilm development in marine environments. Microbiol Mol Biol Rev, 80: 91138
Dang H Y, Luan X W, Chen R P, Zhang X X, Guo L Z, Klotz M G. 2010. Diversity, abundance and distribution of amoA-encoding archaea in deep-sea methane seep sediments of the Okhotsk Sea. Fems Microbiol Ecol, 72: 370-385
Dang H Y, Luan X W, Zhao J Y, Li J. 2009. Diverse and novel nifH and nfH-like gene sequences in the deep-sea methane seep sediments of the Okhotsk Sea. Appl Environ Microbiol, 75: 2238-2245
Dang H Y, Zhou H X, Yang J Y, Ge H M, Jiao N Z, Luan X W, Zhang C L, Klotz M G. 2013. Thaumarchaeotal signature gene distribution in sediments of the northern South China Sea: An indicator of the metabolic intersection of the marine carbon, nitrogen, and phosphorus cycles? Appl Environ Microbiol, 79: 2137-2147
Dang H, Jiao N. 2014. Perspectives on the microbial carbon pump with special reference to microbial respiration and ecosystem efficiency in large estuarine systems. Biogeosciences, 11: 3887-3898
de Angelis M A, Lee C. 1994. Methane production during zooplankton grazing on marine phytoplankton. Limnol Oceanogr, 39: 1298-1308
Dedysh S N, Ricke P, Liesack W. 2004. NifH and NifD phylogenies: An evolutionary basis for understanding nitrogen fixation capabilities of methanotrophic bacteria. Microbiology, 150: 1301-1313
Dekas A E, Poretsky R S, Orphan V J. 2009. Deep-sea archaea fix and share nitrogen in methane-consuming microbial consortia. Science, 326: 422-426
del Valle D, Karl D. 2014. Aerobic production of methane from dissolved water-column methylphosphonate and sinking particles in the North
Pacific Subtropical Gyre. Aquat Microb Ecol, 73: 93-105
DeVries T, Holzer M, Primeau F. 2017. Recent increase in oceanic carbon uptake driven by weaker upper-ocean overturning. Nature, 542: 215218
Dickens G R. 2011. Down the Rabbit Hole: Toward appropriate discussion of methane release from gas hydrate systems during the Paleocene- Eocene thermal maximum and other past hyperthermal events. Clim Past, 7: 831-846
Dickens G R, Castillo M M, Walker J C G. 1997. A blast of gas in the latest Paleocene: Simulating first-order effects of massive dissociation of oceanic methane hydrate. Geology, 25: 259-262
Dickens G R, O'Neil J R, Rea D K, Owen R M. 1995. Dissociation of oceanic methane hydrate as a cause of the carbon isotope excursion at the end of the Paleocene. Paleoceanography, 10: 965-971
Dickinson R E, Cicerone R J. 1986. Future global warming from atmospheric trace gases. Nature, 319: 109-115
DiSpirito A A, Semrau J D, Murrell J C, Gallagher W H, Dennison C, Vuilleumier S. 2016. Methanobactin and the link between copper and bacterial methane oxidation. Microbiol Mol Biol Rev, 80: 387-409
Ditchfield A, Wilson S, Hart M, Purdy K, Green D, Hatton A. 2012. Identification of putative methylotrophic and hydrogenotrophic methanogens within sedimenting material and copepod faecal pellets. Aquat Microb Ecol, 67: 151-160
Dlugokencky E J, Nisbet E G, Fisher R, Lowry D. 2011. Global atmospheric methane: Budget, changes and dangers. Philos Trans R Soc A- Math Phys Eng Sci, 369: 2058-2072
Dyhrman S T, Benitez-Nelson C R, Orchard E D, Haley S T, Pellechia P J. 2009. A microbial source of phosphonates in oligotrophic marine systems. Nat Geosci, 2: 696-699
Dziewit L, Pyzik A, Romaniuk K, Sobczak A, Szczesny P, Lipinski L, Bartosik D, Drewniak L. 2015. Novel molecular markers for the detection of methanogens and phylogenetic analyses of methanogenic communities. Front Microbiol, 6: 694
Embley R W, Chadwick W W, Baker E T, Butterfield D A, Resing J A, de Ronde C E J, Tunnicliffe V, Lupton J E, Juniper S K, Rubin K H, Stern R J, Lebon G T, Nakamura K I, Merle S G, Hein J R, Wiens D A, Tamura Y. 2006. Long-term eruptive activity at a submarine arc volcano. Nature, 441: 494-497
Etminan M, Myhre G, Highwood E J, Shine K P. 2016. Radiative forcing of carbon dioxide, methane, and nitrous oxide: A significant revision of the methane radiative forcing. Geophys Res Lett, 43: 12,614-12,623
Evans P N, Parks D H, Chadwick G L, Robbins S J, Orphan V J, Golding S D, Tyson G W. 2015. Methane metabolism in the archaeal phylum Bathyarchaeota revealed by genome-centric metagenomics. Science, 350: 434-438
Farias L, Sanzana K, Sanhueza-Guevara S, Yevenes M A. 2017. Dissolved methane distribution in the Reloncavi Fjord and adjacent marine system during austral winter (41 -43 S). Estuar Coast, 40: 1592-1606
Fernandez-Carrera A, Rogers K L, Weber S C, Chanton J P, Montoya J P. 2016. Deep Water Horizon oil and methane carbon entered the food web in the Gulf of Mexico. Limnol Oceanogr, 61: S387-S400
Ferry J G, Lessner D J. 2008. Methanogenesis in marine sediments. Ann New York Acad Sci, 1125: 147-157
Finster K, Tanimoto Y, Bak F. 1992. Fermentation of methanethiol and dimethylsulfide by a newly isolated methanogenic bacterium. Arch Microbiol, 157: 425-430
Fischer D, Mogollon J M, Strasser M, Pape T, Bohrmann G, Fekete N, Spiess V, Kasten S. 2013. Subduction zone earthquake as potential trigger of submarine hydrocarbon seepage. Nat Geosci, 6: 647-651
Florez-Leiva L, Damm E, Farias L. 2013. Methane production induced by dimethylsulfide in surface water of an upwelling ecosystem. Prog Oceanogr, 112-113: 38-48
Forster P, Ramaswamy V, Artaxo P, Berntsen T, Betts R, Fahey D W, Haywood J, Lean J, Lowe D C, Myhre G, Nganga J, Prinn R, Raga G, Schulz M, Dorland R V. 2007. Changes in atmospheric constituents and in radiative forcing. Chapter 2. In: Solomon S, Qin D, Manning M, Marquis M, Averyt K, Tignor M M, Miller H L, eds. Climate Change 2007一The Physical Science Basis. Contribution of Working Group I to the Fourth Assessment Report of the Intergovernmental Panel on Climate Change. New York: Cambridge University Press
Friedrich M W. 2005. Methyl-coenzyme M reductase genes: Unique functional markers for methanogenic and anaerobic methane-oxidizing Archaea. Methods Enzymol, 397: 428-442
Frondel M, Oertel K, Rubbelke D. 2002. The domino effect in climate change. Int J Environ Poll, 17: 201-210
Fu H, Metcalf W W. 2015. Genetic basis for metabolism of methylated sulfur compounds in Methanosarcina species. J Bacteriol, 197: 1515- 1524
Garcia J L, Patel B K C, Ollivier B. 2000. Taxonomic, phylogenetic, and ecological diversity of methanogenic Archaea. Anaerobe, 6: 205-226
Geersen J, Scholz F, Linke P, Schmidt M, Lange D, Behrmann J H, Volker D, Hensen C. 2016. Fault zone controlled seafloor methane seepage in the rupture area of the 2010 Maule earthquake, Central Chile. Geochem Geophys Geosyst, 17: 4802-4813
Geissler W H, Gebhardt A C, Gross F, Wollenburg J, Jensen L, Schmidt- Aursch M C, Krastel S, Elger J, Osti G. 2016. Arctic megaslide at presumed rest. Sci Rep, 6: 38529
Gilly W F, Beman J M, Litvin S Y, Robison B H. 2013. Oceanographic and biological effects of shoaling of the oxygen minimum zone. Annu Rev Mar Sci, 5: 393-420
Glasby G P. 2003. Potential impact on climate of the exploitation of methane hydrate deposits offshore. Mar Pet Geol, 20: 163-175
Ghosh A, Patra P K, Ishijima K, Umezawa T, Ito A, Etheridge D M, Sugawara S, Kawamura K, Miller J B, Dlugokencky E J, Krummel P B, Fraser P J, Steele L P, Langenfelds R L, Trudinger C M, White J W C, Vaughn B, Saeki T, Aoki S, Nakazawa T. 2015. Variations in global methane sources and sinks during 1910-2010. Atmos Chem Phys, 15: 2595-2612
Glass J B, Orphan V J. 2012. Trace metal requirements for microbial enzymes involved in the production and consumption of methane and nitrous oxide. Front Microbio, 3: 61
Greening C, Ahmed F H, Mohamed A E, Lee B M, Pandey G, Warden A C, Scott C, Oakeshott J G, Taylor M C, Jackson C J. 2016. Physiology, Biochemistry, and Applications of F420- and F -Dependent Redox Reactions. Microbiol Mol Biol Rev, 80: 451-493
Gruber N. 2011. Warming up, turning sour, losing breath: Ocean biogeochemistry under global change. Philos Trans R Soc A-Math Phys Eng Sci, 369: 1980-1996
Gutjahr M, Ridgwell A, Sexton P F, Anagnostou E, Pearson P N, Palike H, Norris R D, Thomas E, Foster G L. 2017. Very large release of mostly volcanic carbon during the Palaeocene-Eocene Thermal Maximum. Nature, 548: 573-577
Hamdan L J, Wickland K P. 2016. Methane emissions from oceans, coasts, and freshwater habitats: New perspectives and feedbacks on climate. Limnol Oceanogr, 61: S3-S12
Handwerger A L, Rempel A W, Skarbek R M. 2017. Submarine landslides triggered by destabilization of high-saturation hydrate anomalies. Geochem Geophys Geosyst, 18: 2429-2445
Hansen J, Kharecha P, Sato M, Masson-Delmotte V, Ackerman F, Beerling D J, Hearty P J, Hoegh-Guldberg O, Hsu S L, Parmesan C, Rockstrom J, Rohling E J, Sachs J, Smith P, Steffen K, Van Susteren L, von Schuckmann K, Zachos J C. 2013. Assessing “dangerous climate change": Required reduction of carbon emissions to protect young people, future generations and nature. Plos One, 8: e81648
Hansen J, Sato M, Kharecha P, Russell G, Lea D W, Siddall M. 2007. Climate change and trace gases. Philos Trans R Soc A-Math Phys Eng Sci, 365: 1925-1954
Hesselbo S P, Grocke D R, Jenkyns H C, Bjerrum C J, Farrimond P, Morgans Bell H S, Green O R. 2000. Massive dissociation of gas hydrate during a Jurassic oceanic anoxic event. Nature, 406: 392-395
Hester K C, Brewer P G. 2009. Clathrate hydrates in nature. Annu Rev Mar Sci, 1: 303-327
Higgins J A, Schrag D P. 2006. Beyond methane: Towards a theory for the Paleocene-Eocene Thermal Maximum. Earth Planet Sci Lett, 245: 523-
537
Holmes M E, Sansone F J, Rust T M, Popp B N. 2000. Methane production, consumption, and air-sea exchange in the open ocean: An evaluation based on carbon isotopic ratios. Glob Biogeochem Cycle, 14: 110
Horsman G P, Zechel D L. 2017. Phosphonate biochemistry. Chem Rev, 117: 5704-5783
Hove-Jensen B, Zechel D L, Jochimsen B. 2014. Utilization of glyphosate as phosphate source: Biochemistry and genetics of bacterial carbonphosphorus lyase. Microbiol Mol Biol Rev, 78: 176-197
Hunter S J, Goldobin D S, Haywood A M, Ridgwell A, Rees J G. 2013. Sensitivity of the global submarine hydrate inventory to scenarios of future climate change. Earth Planet Sci Lett, 367: 105-115
lino T, Tamaki H, Tamazawa S, Ueno Y, Ohkuma M, Suzuki K, Igarashi Y, Haruta S. 2013. Candidates Methanogranum caenicola: A novel methanogen from the anaerobic digested sludge, and proposal of Metha- nomassiliicoccaceae fam. nov. and Methanomassiliicoccales ord. nov., for a methanogenic lineage of the class Thermoplasmata. Microb Environ, 28: 244-250
Isaksen ISA, Gauss M, Myhre G, Walter Anthony K M, Ruppel C. 2011. Strong atmospheric chemistry feedback to climate warming from Arctic methane emissions. Glob Biogeochem Cycle, 25: GB2002
Jablonski S, Rodowicz P, Lukaszewicz M. 2015. Methanogenic archaea database containing physiological and biochemical characteristics. Int J Systatic Evolary Microbiol, 65: 1360-1368
James R H, Bousquet P, Bussmann I, Haeckel M, Kipfer R, Leifer I, Niemann H, Ostrovsky I, Piskozub J, Rehder G, Treude T, Vielstadte L, Greinert J. 2016. Effects of climate change on methane emissions from seafloor sediments in the Arctic Ocean: A review. Limnol Oceanogr, 61: S283-S299
Jarrell K F. 1985. Extreme oxygen sensitivity in methanogenic archae- bacteria. BioScience, 35: 298-302
Jiao N, Robinson C, Azam F, Thomas H, Baltar F, Dang H, Hardman- Mountford N J, Johnson M, Kirchman D L, Koch B P, Legendre L, Li C, Liu J, Luo T, Luo Y W, Mitra A, Romanou A, Tang K, Wang X, Zhang C, Zhang R. 2014. Mechanisms of microbial carbon sequestration in the ocean-future research directions. Biogeosciences, 11: 52855306
Kallistova A Y, Merkel A Y, Tarnovetskii I Y, Pimenov N V. 2017. Methane formation and oxidation by prokaryotes. Microbiology, 86: 671691
Karl D M, BeversdorfL, Bjorkman K M, Church M J, Martinez A, Delong E F. 2008. Aerobic production of methane in the sea. Nat Geosci, 1: 473-478
Karl D M, Tilbrook B D. 1994. Production and transport of methane in oceanic particulate organic matter. Nature, 368: 732-734
Karol' I L, Kiselev A A, Genikhovich E L, Chicherin S S. 2013. Reduction of short-lived atmospheric pollutant emissions as an alternative strategy for climate-change moderation. Izv Atmos Ocean Phys, 49: 461-478
Karthikeyan O P, Chidambarampadmavathy K, Cires S, Heimann K. 2015. Review of sustainable methane mitigation and biopolymer production. Critical Rev Environ Sci Tech, 45: 1579-1610
Katz M E, Pak D K, Dickens G R, Miller K G. 1999. The source and fate of massive carbon input during the latest Paleocene Thermal Maximum. Science, 286: 1531-1533
Kennedy M, Mrofka D, von der Borch C. 2008. Snowball Earth termination by destabilization of equatorial permafrost methane clathrate. Nature, 453: 642-645
Kennett J P, Cannariato K G, Hendy I L, Behl R J. 2003. Methane hydrates in Quaternary climate change: The clathrate gun hypothesis. AGU, Washington D C. 217
Khadem A F, Pol A, Jetten M S M, Op den Camp H J M. 2010. Nitrogen fixation by the verrucomicrobial methanotroph ‘Methylacidiphilum fu- mariolicum1 SolV Microbiology, 156: 1052-1059
Kiene R P, Oremland R S, Catena A, Miller L G, Capone D G. 1986. Metabolism of reduced methylated sulfur compounds in anaerobic sediments and by a pure culture of an estuarine methanogen. Appl En-
viron Microbiol, 52: 1037-1045
Kirschke S, Bousquet P, Ciais P, Saunois M, Canadell J G, Dlugokencky E J, Bergamaschi P, Bergmann D, Blake D R, Bruhwiler L, Cameron- Smith P, Castaldi S, Chevallier F, Feng L, Fraser A, Heimann M, Hodson E L, Houweling S, Josse B, Fraser P J, Krummel P B, La- marque J F, Langenfelds R L, Le Quere C, Naik V, O'Doherty S, Palmer P I, Pison I, Plummer D, Poulter B, Prinn R G, Rigby M, Ringeval B, Santini M, Schmidt M, Shindell D T, Simpson I J, Spahni R, Steele L P, Strode S A, Sudo K, Szopa S, van der Werf G R, Voulgarakis A, van Weele M, Weiss R F, Williams J E, Zeng G. 2013. Three decades of global methane sources and sinks. Nat Geosci, 6: 813823
Knittel K, Boetius A. 2009. Anaerobic oxidation of methane: Progress with an unknown process. Annu Rev Microbiol, 63: 311-334
Kretschmer K, Biastoch A, Rupke L, Burwicz E. 2015. Modeling the fate of methane hydrates under global warming. Glob Biogeochem Cycle, 29: 610-625
Krishnakumar A M, Sliwa D, Endrizzi J A, Boyd E S, Ensign S A, Peters J W. 2008. Getting a handle on the role of coenzyme M in alkene metabolism. Microbiol Mol Biol Rev, 72: 445-456
Kruger M, Treude T, Wolters H, Nauhaus K, Boetius A. 2005. Microbial methane turnover in different marine habitats. Palaeogeogr Palaeocli- matol Palaeoecol, 227: 6-17
Krumhardt K M, Lovenduski N S, Iglesias-Rodriguez M D, Kleypas J A. 2017. Coccolithophore growth and calcification in a changing ocean. Prog Oceanogr, 159: 276-295
Kudela R M, Seeyave S, Cochlan W P. 2010. The role of nutrients in regulation and promotion of harmful algal blooms in upwelling systems. Prog Oceanogr, 85: 122-135
Kvenvolden K A. 1988. Methane hydrates and global climate. Glob Bio- geochem Cycle, 2: 221-229
Lambert G, Schmidt S. 1993. Reevaluation of the oceanic flux of methane: Uncertainties and long term variations. Chemosphere, 26: 579-589
Lamontagne R A, Swinnerton J W, Linnenbom V J. 1971. Nonequilibrium of carbon monoxide and methane at the air-sea interface. J Geophys Res, 76: 5117-5121
Leifer I, Luyendyk B P, Boles J, Clark J F. 2006. Natural marine seepage blowout: Contribution to atmospheric methane. Glob Biogeochem Cycle, 20: GB3008
Lenhart K, Klintzsch T, Langer G, Nehrke G, Bunge M, Schnell S, Keppler F. 2016. Evidence for methane production by the marine algae Emi- liania huxleyi. Biogeosciences, 13: 3163-3174
Levin L A, Le Bris N. 2015. The deep ocean under climate change. Science, 350: 766-768
Liu Y, Whitman W B. 2008. Metabolic, phylogenetic, and ecological diversity of the methanogenic archaea. Ann New York Acad Sci, 1125: 171-189
Lloyd K. 2015. Beyond known methanogens. Science, 350: 384
Lomans B P, Maas R, Luderer R, Op den Camp H J, Pol A, van der Drift C, Vogels G D. 1999. Isolation and characterization of Methanomethylo- vorans hollandica gen. nov., sp. nov., isolated from freshwater sediment, a methylotrophic methanogen able to grow on dimethyl sulfide and methanethiol. Appl Environ Microbiol, 65: 3641-3650
Loulergue L, Schilt A, Spahni R, Masson-Delmotte V, Blunier T, Lemieux B, Barnola J M, Raynaud D, Stocker T F, Chappellaz J. 2008. Orbital and millennial-scale features of atmospheric CH4 over the past 800000 years. Nature, 453: 383-386
Lyimo T J, Pol A, Op den Camp H J, Harhangi H R, Vogels G D. 2000. Methanosarcina semesiae sp. nov., a dimethylsulfide-utilizing methanogen from mangrove sediment. Int J Systatic Evolary Microbiol, 50: 171-178
Lyu Z, Lu Y. 2018. Metabolic shift at the class level sheds light on adaptation of methanogens to oxidative environments. ISME J, 12: 411423
MacCracken M C. 2008. Prospects for future climate change and the reasons for early action. J Air Waste Manage, 58: 735-786
MacDougall A H, Knutti R. 2016. Enhancement of non-CO? radiative
forcing via intensified carbon cycle feedbacks. Geophys Res Lett, 43: 5833-5840
McKinley G A, Pilcher D J, Fay A R, Lindsay K, Long M C, Lovenduski N S. 2016. Timescales for detection of trends in the ocean carbon sink. Nature, 530: 469X72
Marin-Moreno H, Giustiniani M, Tinivella U, Pinero E. 2016. The challenges of quantifying the carbon stored in Arctic marine gas hydrate. Mar Pet Geol, 71: 76-82
Marin-Moreno H, Minshull T A, Westbrook G K, Sinha B, Sarkar S. 2013. The response of methane hydrate beneath the seabed offshore Svalbard to ocean warming during the next three centuries. Geophys Res Lett, 40: 5159-5163
Martinez A, Ventouras L A, Wilson S T, Karl D M, DeLong E F. 2013. Metatranscriptomic and functional metagenomic analysis of methylphosphonate utilization by marine bacteria. Front Microbiol, 4: 340
Marty D G, Nival P, Yoon W D. 1997. Methanoarchaea associated with sinking particles and zooplankton collected in the Northeastern tropical Atlantic. Oceanol Acta, 20: 863-869
Maslin M, Owen M, Betts R, Day S, Dunkley Jones T, Ridgwell A. 2010. Gas hydrates: Past and future geohazard? Philos Trans R Soc A-Math Phys Eng Sci, 368: 2369-2393
Maslin M, Owen M, Day S, Long D. 2004. Linking continental-slope failures and climate change: Testing the clathrate gun hypothesis. Geology, 32: 53-56
Masson D G, Harbitz C B, Wynn R B, Pedersen G, Lovholt F. 2006. Submarine landslides: Processes, triggers and hazard prediction. Philos Trans R Soc A-Math Phys Eng Sci, 364: 2009-2039
Masuda S, Awaji T, Sugiura N, Matthews J P, Toyoda T, Kawai Y, Doi T, Kouketsu S, Igarashi H, Katsumata K, Uchida H, Kawano T, Fukasawa M. 2010. Simulated rapid warming of abyssal North Pacific waters. Science, 329: 319-322
Matthews E. 1994. Assessment of methane sources and their uncertainties. Pure Appl Chem, 66: 154-162
McNeall D, Halloran P R, Good P, Betts R A. 2011. Analyzing abrupt and nonlinear climate changes and their impacts. WIREs Clim Change, 2: 663-686
Mestdagh T, Poort J, De Batist M. 2017. The sensitivity of gas hydrate reservoirs to climate change: Perspectives from a new combined model for permafrost-related and marine settings. Earth-Sci Rev, 169: 104131
Metcalf W W, Griffin B M, Cicchillo R M, Gao J, Janga S C, Cooke H A, Circello B T, Evans B S, Martens-Habbena W, Stahl D A, van der Donk W A. 2012. Synthesis of methylphosphonic acid by marine microbes: A source for methane in the aerobic ocean. Science, 337: 1104-1107
Mondav R, Woodcroft B J, Kim E H, McCalley C K, Hodgkins S B, Crill P M, Chanton J, Hurst G B, VerBerkmoes N C, Saleska S R, Hugenholtz
P, Rich V I, Tyson G W. 2014. Discovery of a novel methanogen prevalent in thawing permafrost. Nat Commun, 5: 3212
Montzka S A, Dlugokencky E J, Butler J H. 2011. Non-CO? greenhouse gases and climate change. Nature, 476: 43-50
Mora C, Wei C L, Rollo A, Amaro T, Baco A R, Billett D, Bopp L, Chen
Q, Collier M, Danovaro R, Gooday A J, Grupe B M, Halloran P R, Ingels J, Jones D O B, Levin L A, Nakano H, Norling K, Ramirez- Llodra E, Rex M, Ruhl H A, Smith C R, Sweetman A K, Thurber A R, Tjiputra J F, Usseglio P, Watling L, Wu T, Yasuhara M. 2013. Biotic and human vulnerability to projected changes in ocean biogeochemistry over the 21st century. Plos Biol, 11: e1001682
Naqvi SWA, Bange H W, Farias L, Monteiro PMS, Scranton M I, Zhang J. 2010. Marine hypoxia/anoxia as a source of CH4 and N?O. Biogeosciences, 7: 2159-2190
Navid D. 1989. The international law of migratory species: The Ramsar convention. Nat Res J, 29: 1001-1016
Nobu M K, Narihiro T, Kuroda K, Mei R, Liu W T. 2016. Chasing the elusive Euryarchaeota class WSA2: Genomes reveal a uniquely fastidious methyl-reducing methanogen. ISME J, 10: 2478-2487
Norris R D, Rohl U. 1999. Carbon cycling and chronology of climate warming during the Palaeocene/Eocene transition. Nature, 401: 775-
778
Obzhirov A I. 2013. Gas component increase during seismo-tectonics and the role of gas in earthquake origination (Okhotsk Sea). Russ J Pac Geol, 32: 86-89
Offre P, Spang A, Schleper C. 2013. Archaea in biogeochemical cycles. Annu Rev Microbiol, 67: 437-457
Orcutt B N, LaRowe D E, Biddle J F, Colwell F S, Glazer B T, Reese B K, Kirkpatrick J B, Lapham L L, Mills H J, Sylvan J B, Wankel S D, Wheat C G. 2013. Microbial activity in the marine deep biosphere: Progress and prospects. Front Microbiol, 4: 189
Oremland R S. 1979. Methanogenic activity in plankton samples and fish intestines A mechanism for in situ methanogenesis in oceanic surface waters. Limnol Oceanogr, 24: 1136-1141
Oremland R S, Kiene R P, Mathrani I, Whiticar M J, Boone D R. 1989. Description of an estuarine methylotrophic methanogen which grows on dimethyl sulfide. Appl Environ Microbiol, 55: 994-1002
Ortiz-Llorente M J, Alvarez-Cobelas M. 2012. Comparison of biogenic methane emissions from unmanaged estuaries, lakes, oceans, rivers and wetlands. Atmos Environ, 59: 328-337
Paulo L M, Ramiro-Garcia J, van Mourik S, Stams A J M, Sousa D Z. 2017. Effect of nickel and cobalt on methanogenic enrichment cultures and role of biogenic sulfide in metal toxicity attenuation. Front Microbiol, 8: 1341
Pernthaler A, Dekas A E, Titus Brown C, Goffredi S K, Embaye T, Orphan V J. 2008. Diverse syntrophic partnerships from deep-sea methane vents revealed by direct cell capture and metagenomics. Proc Natl Acad Sci USA, 105: 7052-7057
Phrampus B J, Hornbach M J. 2012. Recent changes to the Gulf Stream causing widespread gas hydrate destabilization. Nature, 490: 527-530
Poehlein A, Daniel R, Seedorf H. 2017. The draft genome of the non-host- associated Methanobrevibacter arboriphilus strain DH1 encodes a large repertoire of adhesin-like proteins. Archaea, 2017: 1-9
Pohlman J W, Greinert J, Ruppel C, Silyakova A, Vielstadte L, Casso M, Mienert J, Bunz S. 2017. Enhanced CO? uptake at a shallow Arctic Ocean seep field overwhelms the positive warming potential of emitted methane. Proc Natl Acad Sci USA, 114: 5355-5360
Prather M J, Holmes C D. 2017. Overexplaining or underexplaining methane's role in climate change. Proc Natl Acad Sci USA, 114: 53245326
Purwantini E, Torto-Alalibo T, Lomax J, Setubal JAC, Tyler B M, Mu- khopadhyay B. 2014. Genetic resources for methane production from biomass described with the Gene Ontology. Front Microbiol, 5: 634
Rakowski C V, Magen C, Bosman S, Rogers K L, Gillies L E, Chanton J P, Mason O U. 2015. Methane and microbial dynamics in the Gulf of Mexico water column. Front Mar Sci, 2: 69
Rasmussen R A, Khalil M A K. 1981. Atmospheric methane (CH4 ): Trends and seasonal cycles. J Geophys Res, 86: 9826-9832
Ravishankara A R, Daniel J S, Portmann R W. 2009. Nitrous oxide (巨0): The dominant ozone-depleting substance emitted in the 21st century. Science, 326: 123-125
Reagan M T, Moridis G J. 2007. Oceanic gas hydrate instability and dissociation under climate change scenarios. Geophys Res Lett, 34: L22709
Reagan M T, Moridis G J. 2008. Dynamic response of oceanic hydrate deposits to ocean temperature change. J Geophys Res, 113: C12023
Reeburgh W S. 2007. Oceanic methane biogeochemistry. Chem Rev, 107: 486-513
Repeta D J, Ferron S, Sosa O A, Johnson C G, Repeta L D, Acker M, DeLong E F, Karl D M. 2016. Marine methane paradox explained by bacterial degradation of dissolved organic matter. Nat Geosci, 9: 884887
Rhee T S, Kettle A J, Andreae M O. 2009. Methane and nitrous oxide emissions from the ocean: A reassessment using basin-wide observations in the Atlantic. J Geophys Res, 114: D12304
Ruppel C D, Kessler J D. 2017. The interaction of climate change and methane hydrates. Rev Geophys, 55: 126-168
Sabine C L, Feely R A, Gruber N, Key R M, Lee K, Bullister J L, Wan- ninkhof R, Wong C S, Wallace DW R, Tilbrook B, Millero F J, Peng T H, Kozyr A, Ono T, Rios A F. 2004. The oceanic sink for anthropogenic CO2. Science, 305: 367-371
Sakai S, Imachi H, Hanada S, Ohashi A, Harada H, Kamagata Y. 2008. Methanocella paludicola gen. nov., sp. nov., a methane-producing archaeon, the first isolate of the lineage ‘Rice Cluster I', and proposal of the new archaeal orderMethanocellales ord. nov.. Int J Systatic Evolary Microbiol, 58: 929-936
Sansone F J, Popp B N, Gasc A, Graham A W, Rust T M. 2001. Highly elevated methane in the eastern tropical North Pacific and associated isotopically enriched fluxes to the atmosphere. Geophys Res Lett, 28: 4567-4570
Sasakawa M, Tsunogai U, Kameyama S, Nakagawa F, Nojiri Y, Tsuda A. 2008. Carbon isotopic characterization for the origin of excess methane in subsurface seawater. J Geophys Res, 113: C03012
Schafer G, Engelhard M, Muller V 1999. Bioenergetics of the Archaea. Microbiol Mol Biol Rev, 63: 570-620
Schink B. 1997. Energetics of syntrophic cooperation in methanogenic degradation. Microbiol Mol Biol Rev, 61: 262-280
Schleuning M, Frund J, Schweiger O, Welk E, Albrecht J, Albrecht M, Beil M, Benadi G, Bluthgen N, Bruelheide H, Bohning-Gaese K, Dehling D M, Dormann C F, Exeler N, Farwig N, Harpke A, Hickler T, Kra- tochwil A, Kuhlmann M, Kuhn I, Michez D, Mudri-Stojnic S, Plein M, Rasmont P, Schwabe A, Settele J, Vujic A, Weiner C N, Wiemers M, Hof C. 2016. Ecological networks are more sensitive to plant than to animal extinction under climate change. Nat Commun, 7: 13965
Schmale O, Wage J, Mohrholz V, Wasmund N, Grawe U, Rehder G, Labrenz M, Loick-Wilde N. 2018. The contribution of zooplankton to methane supersaturation in the oxygenated upper waters of the central Baltic Sea. Limnol Oceanogr, 63: 412-430
Scranton M I, Brewer P G. 1977. Occurrence of methane in the nearsurface waters of the western subtropical North-Atlantic. Deep Sea Res, 24: 127-138
Sela-Adler M, Ronen Z, Herut B, Antler G, Vigderovich H, Eckert W, Sivan O. 2017. Co-existence of methanogenesis and sulfate reduction with common substrates in sulfate-rich estuarine sediments. Front Microbiol, 8: 766
Semrau J D, DiSpirito A A, Gu W, Yoon S. 2018. Metals and methano- trophy. Appl Environ Microbiol, 84: e02289-17
Shakhova N, Semiletov I, Salyuk A, Yusupov V, Kosmach D, Gustafsson O. 2010. Extensive methane venting to the atmosphere from sediments of the East Siberian Arctic Shelf. Science, 327: 1246-1250
Shepherd J G, Brewer P G, Oschlies A, Watson A J. 2017. Ocean ventilation and deoxygenation in a warming world: Introduction and overview. Philos Trans R Soc A-Math Phys Eng Sci, 375: 20170240
Showstack R. 2013. Carbon Dioxide Tops 400 ppm at Mauna Loa. Hawaii: Eos Trans AGU, 94: 192
Sieburth J N, Johnson P, Macario A, Conway de Macario E. 1993. C1 bacteria in the water column of Chesapeake Bay USA. II. The dominant O2- and H2S-tolerant methylotrophic methanogens, coenriched with their oxidative and sulphate reducing bacterial consorts, are all new immunotypes and probably include new taxa. Mar Ecol Prog Ser, 95: 81-89
Solomon S, Daniel J S, Sanford T J, Murphy D M, Plattner G K, Knutti R, Friedlingstein P. 2010. Persistence of climate changes due to a range of greenhouse gases. Proc Natl Acad Sci USA, 107: 18354-18359
Solomon S, Plattner G K, Knutti R, Friedlingstein P. 2009. Irreversible climate change due to carbon dioxide emissions. Proc Natl Acad Sci USA, 106: 1704-1709
Sonnemann G R, Grygalashvyly M. 2014. Global annual methane emission rate derived from its current atmospheric mixing ratio and estimated lifetime. Ann Geophys, 32: 277-283
Sorokin D Y, Makarova K S, Abbas B, Ferrer M, Golyshin P N, Galinski E A, Ciordia S, Mena M C, Merkel A Y, Wolf Y I, van Loosdrecht M C M, Koonin E V. 2017. Discovery of extremely halophilic, methyl-re- ducing euryarchaea provides insights into the evolutionary origin of methanogenesis. Nat Microbiol, 2: 17081
Sosa O A, Repeta D J, Ferron S, Bryant J A, Mende D R, Karl D M, DeLong E F. 2017. Isolation and characterization of bacteria that degrade phosphonates in marine dissolved organic matter. Front Microbiol, 8: 1786
Sowers T. 2006. Late Quaternary atmospheric CH4 isotope record suggests marine clathrates are stable. Science, 311: 838-840
Stranne C, O'Regan M, Jakobsson M. 2017. Modeling fracture propagation and seafloor gas release during seafloor warming-induced hydrate dissociation. Geophys Res Lett, 44: 8510-8519
Svensen H, Planke S, Malthe-Sorenssen A, Jamtveit B, Myklebust R, Rasmussen Eidem T, Rey S S. 2004. Release of methane from a volcanic basin as a mechanism for initial Eocene global warming. Nature, 429: 542-545
Sydeman W J, Garcia-Reyes M, Schoeman D S, Rykaczewski R R, Thompson S A, Black B A, Bograd S J. 2014. Climate change and wind intensification in coastal upwelling ecosystems. Science, 345: 77-80
Tallant T C, Krzycki J A. 1997. Methylthiol:coenzyme M methyltransferase from Methanosarcina barkeri, an enzyme of methanogenesis from dimethylsulfide and methylmercaptopropionate. J Bacteriol, 179: 69026911
Tallant T C, Paul L, Krzycki J A. 2001. The MtsA subunit of the me- thylthiol:coenzyme M methyltransferase of Methanosarcina barkeri catalyses both half-reactions of corrinoid-dependent dimethylsulfide: Coenzyme M methyl transfer. J Biol Chem, 276: 4485-4493
Teikari J E, Fewer D P, Shrestha R, Hou S, Leikoski N, Makela M, Si- mojoki A, Hess W R, Sivonen K. 2018. Strains of the toxic and bloomforming Nodularia spumigena (cyanobacteria) can degrade methylphosphonate and release methane. ISME J, 12: 1619-1630
Thatcher K E, Westbrook G K, Sarkar S, Minshull T A. 2013. Methane release from warming-induced hydrate dissociation in the West Svalbard continental margin: Timing, rates, and geological controls. J Geophys Res-Solid Earth, 118: 22-38
Tholen A, Pester M, Brune A. 2007. Simultaneous methanogenesis and oxygen reduction by Methanobrevibacter cuticularis at low oxygen fluxes. Fems Microbiol Ecol, 62: 303-312
Tilbrook B D, Karl D M. 1995. Methane sources, distributions and sinks from California coastal waters to the oligotrophic North Pacific gyre. Mar Chem, 49: 51-64
Tseng H C, Chen C T A, Borges A V, DelValls T A, Chang Y C. 2017. Methane in the South China Sea and the Western Philippine Sea. Cont Shelf Res, 135: 23-34
Tsunogai U, Maegawa K, Sato S, Komatsu D D, Nakagawa F, Toki T, Ashi J. 2012. Coseimic massive methane release from a submarine mud volcano. Earth Planet Sci Lett, 341-344: 79-85
Tsuruta A, Aalto T, Backman L, Hakkarainen J, van der Laan-Luijkx I T, Krol M C, Spahni R, Houweling S, Laine M, Dlugokencky E, Gomez- Pelaez A J, van der Schoot M, Langenfelds R, Ellul R, Arduini J, Apadula F, Gerbig C, Feist D G, Kivi R, Yoshida Y, Peters W. 2017. Global methane emission estimates for 2000-2012 from CarbonTracker Europe-CH4 v1.0. Geosci Model Dev, 10: 1261-1289
Upstill-Goddard R C, Barnes J. 2016. Methane emissions from UK estuaries: Re-evaluating the estuarine source of tropospheric methane from Europe. Mar Chem, 180: 14-23
Valentine D L. 2011. Emerging topics in marine methane biogeochemistry. Annu Rev Mar Sci, 3: 147-171
van der Maarel M J E C, Hansen T A. 1997. Dimethylsulfoniopropionate in anoxic intertidal sediments: A precursor of methanogenesis via dimethyl sulfide, methanethiol, and methiolpropionate. Mar Geol, 137: 512
Van Mooy B A S, Krupke A, Dyhrman S T, Fredricks H F, Frischkorn K R, Ossolinski J E, Repeta D J, Rouco M, Seewald J D, Sylva S P. 2015. Major role of planktonic phosphate reduction in the marine phosphorus redox cycle. Science, 348: 783-785
Vanwonterghem I, Evans P N, Parks D H, Jensen P D, Woodcroft B J, Hugenholtz P, Tyson G W. 2016. Methylotrophic methanogenesis discovered in the archaeal phylum Verstraetearchaeota. Nat Microbiol, 1: 16170
Vizza C, West W E, Jones S E, Hart J A, Lamberti G A. 2017. Regulators of coastal wetland methane production and responses to simulated global change. Biogeosciences, 14: 431-446
Vojvoda J, Lamy D, Sintes E, Garcia J, Turk V, Herndl G. 2014. Seasonal variation in marine-snow-associated and ambient-water prokaryotic communities in the northern Adriatic Sea. Aquat Microb Ecol, 73: 211- 224
Wang D, Gouhier T C, Menge B A, Ganguly A R. 2015. Intensification and spatial homogenization of coastal upwelling under climate change. Nature, 518: 390-394
Wang J, Yuan J, Liu D, Xiang J, Ding W, Jiang X. 2016. Research progresses on methanogenesis pathway and methanogens in coastal wetlands. Chin J Appl Ecol, 27: 993-1001
Weller D I, Law C S, Marriner A, Nodder S D, Chang F H, Stephens J A, Wilhelm S W, Boyd P W, Sutton P J H. 2013. Temporal variation of dissolved methane in a subtropical mesoscale eddy during a phytoplankton bloom in the southwest Pacific Ocean. Prog Oceanogr, 116: 193-206
Welsh D T. 2000. Ecological significance of compatible solute accumulation by micro-organisms: From single cells to global climate. Fems Microbiol Rev, 24: 263-290
Welte C, Deppenmeier U. 2014. Bioenergetics and anaerobic respiratory chains of aceticlastic methanogens. Biochim Biophysica Acta, 1837: 1130-1147
Wen X, Yang S, Horn F, Winkel M, Wagner D, Liebner S. 2017. Global biogeographic analysis of methanogenic archaea identifies communityshaping environmental factors of natural environments. Front Microbiol, 8: 1339
Wilson S T, Ferron S, Karl D M. 2017. Interannual variability of methane and nitrous oxide in the North Pacific Subtropical Gyre. Geophys Res Lett, 44: 9885-9892
Wright J J, Konwar K M, Hallam S J. 2012. Microbial ecology of expanding oxygen minimum zones. Nat Rev Micro, 10: 381-394
Wuebbles D J, Hayhoe K. 2002. Atmospheric methane and global change. Earth-Sci Rev, 57: 177-210
Xiao K Q, Beulig F, Kjeldsen K U, Jorgensen B B, Risgaard-Petersen N. 2017. Concurrent methane production and oxidation in surface sediment
from Aarhus Bay, Denmark. Front Microbiol, 8: 1198
Xiao L, Xie B, Liu J, Zhang H, Han G, Wang O, Liu F. 2017. Stimulation of long-term ammonium nitrogen deposition on methanogenesis by Methanocellaceae in a coastal wetland. Sci Total Environ, 595: 337-343 Yvon-Durocher G, Allen A P, Bastviken D, Conrad R, Gudasz C, St-Pierre A, Thanh-Duc N, del Giorgio P A. 2014. Methane fluxes show consistent temperature dependence across microbial to ecosystem scales. Nature, 507: 488-491
Zeebe R E, Ridgwell A, Zachos J C. 2016. Anthropogenic carbon release rate unprecedented during the past 66 million years. Nat Geosci, 9: 325329
Zehnder A J B, Wuhrmann K. 1977. Physiology of a Methanobacterium strain AZ. Arch Microbiol, 111: 199-205
Zhang B, Tian H, Lu C, Chen G, Pan S, Anderson C, Poulter B. 2017. Methane emissions from global wetlands: An assessment of the uncertainty associated with various wetland extent data sets. Atmos Environ, 165: 310-321
Zhang G L, Zhang J, Kang Y B, Liu S M. 2004. Distributions and fluxes of methane in the East China Sea and the Yellow Sea in spring. J Geophys Res, 109: C07011
Zhang G L, Zhang J, Liu S, Ren J, Xu J, Zhang F. 2008. Methane in the Changjiang (Yangtze River) Estuary and its adjacent marine area: Riverine input, sediment release and atmospheric fluxes. Biogeochemistry, 91: 71-84
Zhang Y, Zhai W D. 2015. Shallow-ocean methane leakage and degassing to the atmosphere: Triggered by offshore oil-gas and methane hydrate explorations. Front Mar Sci, 2: 34
Zhou H Y, Yin X J, Yang Q H, Wang H, Wu Z J, Bao S X. 2009. Distribution, source and flux of methane in the western Pearl River Estuary and northern South China Sea. Mar Chem, 117: 21-31
Zickfeld K, Solomon S, Gilford D M. 2017. Centuries of thermal sea-level rise due to anthropogenic emissions of short-lived greenhouse gases. Proc Natl Acad Sci USA, 114: 657-662
Zindler C, Bracher A, Marandino C A, Taylor B, Torrecilla E, Kock A, Bange H W. 2013. Sulphur compounds, methane, and phytoplankton: Interactions along a north-south transit in the western Pacific Ocean. Biogeosciences, 10: 3297-3311
(Responsible editor: Xiaoxue WANG)