Sulfur metabolism by marine heterotrophic bacteria involved in sulfur cycling in the ocean
Xin HU1, Jihua LIU1*, Huaiwei LIU2, Guangchao ZHUANG3 & Luying XUN2
1 Institute of Marine Science and Technology, Shandong University, Qingdao 266237, China;
2 State Key Laboratory of Microbial Technology, Shandong University, Qingdao 266237, China;
3 Department of Marine Sciences, University of Georgia, Athens, GA 30602, USA
Received November 4, 2017; revised April 10, 2018; accepted June 11, 2018; published online August 8, 2018
Abstract
Sulfur cycling in the biosphere is tightly interwoven with the cycling of carbon and nitrogen, through various biological and geochemical processes. Marine microorganisms, due to their high abundance, diverse metabolic activities, and tremendous adaptation potential, play an essential role in the functioning of global biogeochemical cycles and linking sulfur transformation to the cycling of carbon and nitrogen. Currently many coastal regions are severely stressed by hypoxic or anoxic conditions, leading to the accumulation of toxic sulfide. A number of recent studies have demonstrated that dissimilatory sulfur oxidation by heterotrophic bacteria can protect marine ecosystems from sulfide toxicity. Sulfur-oxidizing bacteria have evolved diverse phylogenetic and metabolic characteristics to fill an array of ecological niches in various marine habitats. Here, we review the recent findings on the microbial communities that are involved in the oxidation of inorganic sulfur compounds and address how the two elements of sulfur and carbon are interlinked and influence the ecology and biogeochemistry in the ocean. Delineating the metabolic enzymes and pathways of sulfur-oxidizing bacteria not only provides an insight into the microbial sulfur metabolism, but also helps us understand the effects of changing environmental conditions on marine sulfur cycling and reinforces the close connection between sulfur and carbon cycling in the ocean.
Keywords Sulfur cycling, Sulfur oxidation, Heterotrophic bacteria, Metabolic pathway
1.Introduction
Sulfur, as an essential element for life, makes up about 1% of the dry weight of organisms. With large quantities of dissolved sulfate and sedimentary minerals in the ocean, sulfur rarely becomes a limiting nutrient; however, its turnover has important consequences for marine ecosystems and the global climate system. Sulfur chemistry is intimately connected with the origin of life on the earth. Long before the dramatic rise in atmospheric oxygen about 600 million years ago, the ocean remained anoxic and severely hypoxic, containing large amounts of sulfur compounds (Li et al., 2015; Xie et al., 2017). Because sulfur is the sixth most abundant element and a major driver in the evolution of life, its metabolism is critically important to global biogeochemical cycles.
Microorganisms are key players in the transformation of both inorganic and organic sulfur compounds in global sulfur cycling (Sievert et al., 2007). Microbial sulfate reduction and sulfur disproportionation result in the formation of substantial amounts of hydrogen sulfide (Jorgensen, 1982). Hydrogen sulfide can also be released from sulfur-containing amino acids, such as cysteine and methionine, during the decomposition of organic matter (Xia et al., 2017). Sulfur-oxidizing bacteria (SOB) can oxidize sulfide to intermediate sulfur species (sulfite and thiosulfate) or oxidize it completely to sulfate, which are energy-yielding reactions, collectively termed dissimilatory sulfur oxidation. This process recycles reduced sulfur compounds back to the oxidized form and thus facilitates their use as electron acceptors by anaerobic microorganisms (Bruser et al., 2000).
In recent years, eutrophication, caused by an excess of mineral nutrients due to the over production of organic matter and the associated severe reduction in dissolved oxygen concentrations, has led to the loss of biodiversity and affected coastal marine ecosystems worldwide (Selman et al., 2008). Currently, seasonal or persistent hypoxia develops in many coastal ecosystems and results in the accumulation of toxic hydrogen sulfide that arises from the sediment through anaerobic carbon mineralization by sulfate reducing microorganisms. The expansion of hypoxic zones could have serious consequences for coastal ecosystems and economies, such asthe loss offisheries, mass mortality, and the alteration of food webs (Diaz and Rosenberg, 2008). However, the energy-yielding oxidation of inorganic sulfur compounds, i.e., dissimilatory sulfur oxidation, by SOB can protect marine organisms from sulfide toxicity (Lavik et al., 2009). The microbial cycling of sulfur and carbon has been the focus of research in marine ecosystems that are severely stressed by hypoxia, including the Baltic Sea (Grote et al., 2008), the Cariaco Basin (Lin et al., 2006) and the Saanich Inlet (Zaikova et al., 2009). It is of fundamental importance to characterize the SOB among prevailing microbial communities to better understand the oxidation and reduction processes that occur during sulfur transformation and predict the effects of changing environmental conditions on cycling of the key elements sulfur and carbon. This review provides an overview of the fate of sulfur and illustrates how the input of organic matter leads to the formation of sulfide, focusing on the metabolic activities and pathways of microbes that mediate the oxidation of inorganic sulfur compounds.
2.Sulfide production in marine ecosystems
The complexity of sulfur cycle originates from the large variety of sulfur oxidation states, ranging from completely reduced (reduction state -2) to completely oxidized (oxidation state +6). Due to the different oxidized and reduced forms, sulfur compounds can be used as electron acceptors in sulfate or sulfur reduction processes, and electron donors in sulfur oxidation processes. The microbe-mediated sulfur cycle is shown in Figure 1.
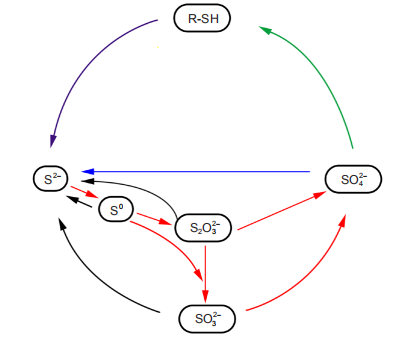
Figure 1 The microbial sulfur cycle. Sulfide is produced by dissimilatory sulfate reduction (blue line) and disproportionation of sulfite, thiosulfate and elemental sulfur (black lines). The purple line depicts the decomposition of sulfur-rich organic matter during which sulfide is also produced. The green line depicts assimilatory sulfate reduction. The red lines depict dissimilatory sulfur oxidation mediated by SOB, which re-oxidizes sulfide to intermediate sulfur species or completely to sulfate.
Sulfate is a ubiquitous electron acceptor in marine environments because of its high abundance and stability in seawater. Sulfate reduction is one of the most important microbial redox processes and is the primary driver of marine sulfur cycling (Bowles et al., 2014). In organic-rich coastal sediments, microbial sulfate reduction accounts for a large proportion of organic matter mineralization and results in the production of substantial amounts of hydrogen sulfide. The reduction of sulfate and sulfite can be either assimilatory for the synthesis of organic sulfur compounds or dissifor the synthesis of organic sulfur compounds or dissim- ilatory to dispose of an excess of reduction equivalents (Leloup et al., 2009). The sulfide produced can be either deposited as metal sulfides, or oxidized to elemental sulfur or sulfate. Furthermore, sulfide can also be derived from the disproportionation reaction of elemental sulfur, sulfite, and thiosulfate (Thamdrup et al., 1993). The inorganic sulfur compounds thiosulfate and sulfite can be disproportionated to hydrogen sulfide and sulfate by numerous genera of sul- fate-reducing bacteria. The disproportionation of elemental sulfur can be attributed to Desufocapsa thiozymogenes, Desufobulbus propionicus 1pr3 and Desulfocapsa sulfoexi- gens sp. nov., which are phylogenetically related and share common phenotypic characteristics (Kai et al., 1998).
Another source of hydrogen sulfide is the process of organic sulfur decomposition termed desulfurization. Marine heterotrophic bacteria can metabolize organic matter, especially sulfur-rich compounds excreted from living phytoplankton cells or released from lysed algal cells. Recently transcriptomics and metagenomics analysis have shown that the organic sulfur compounds dimethylsulfoniopropionate (DMSP) and 2,3-dihydroxypropane-1-sulfonate (DHPS) are key currencies in the trophic interactions (Howard et al., 2008; Durham et al., 2015). The organic sulfur compounds can be degraded to methanethiol (CH3SH; MeSH) and further metabolized to methionine, ultimately releasing H2S from sulfur-containing amino acids cysteine and methionine (Xia et al., 2017). The Roseobacter lineage, with its ubiquitous distribution and versatile metabolic activities, has been found to play a key role in the transformation of organic sulfur compounds (Gonzalez et al., 1999).
The sulfide produced is re-oxidized to intermediate sulfur species (sulfite and thiosulfate) or completely oxidized to sulfate by SOB. This dissimilatory sulfur oxidation process recycles reduced sulfur compounds back to their oxidized form (Lenk, 2011). Some sulfur compounds, notably S2- and HS-, carry out spontaneous chemical reactions with oxygen and heavy metal ions. In many cases, the biological oxidation of sulfur compounds by SOB competes successfully with chemical reactions, in particular with the precipitation of metal sulfides (Jorgensen and Nelson, 2004). Mostly, the reaction rates of biological oxidation far exceed those of chemical oxidation of sulfide according to thermodynamic and kinetic estimations (Luther et al., 2011).
3.Microbial communities of sulfur-oxidizing bacteria
3.1 Diversity of sulfur-oxidizing bacteria
Sulfur-oxidizing bacteria (SOB) are phylogenetically and physiologically diverse, comprising autotrophic SOB (pho- totrophic and chemotrophic SOB), as well as heterotrophic SOB. The anoxygenic phototrophic bacteria consist of five different major phylogenetic lineages: (1) the filamentous anoxygenic phototrophs (FAP; relatives of Chloroflexus), (2) the green sulfur bacteria (GSB; comprising the family Chlorobiaceae and the genus Chloroflexus), (3) the heliobacteria (comprising the family Heliobacteriaceae), (4) the purple bacteria (comprising purple sulfur bacteria within the class of Gammaproteobacteria, which contains the two families Chromatiaceae and Ectothiorhodospiraceae, and purple non-sulfur bacteria within the classes of Alpha- and Beta-proteobacteria), and (5) the phototrophic acidobacteria within the Acidobacteria phylum (Frigaard and Dahl, 2009). Anaerobic phototrophic bacteria can use the reducing power of sulfide for carbon dioxide fixation and bacterial anoxygenic photosynthetic growth (Gregersen et al., 2011).
Chemotrophic SOB, also known as colorless SOB, mainly comprise two morphologically and taxonomically distinct groups: the genus Thiobacillus (Thiobacillus ferrooxidans, Thiobacillus thioparus, Thiobacillus denitrificans, Thiobacillus novellus, and Thiobacillus thiooxidans) and the genera Beggiatoa and Thiothrix. These bacteria are Gram-negative, and differ greatly, even within the same genus. The autotrophic denitrifying SOB can use the oxidized forms of nitrogen (nitrates or nitrites) as electron acceptors for energy conservation and bacterial growth (Lavik et al., 2009; Mangold et al., 2011), mainly including Thiobacillus deni-trificans, Thiomicrospira denitrificans, Thiobacillus versu- tus, and Thiosphaera pantotropha. These bacteria are able to derive energy from the oxidation of a variety of reduced inorganic sulfur species (S2-, S0, SO^-) for their chemo- trophic growth. The final products of sulfide oxidation are elemental sulfur or sulfates and nitrogen gas or nitrites as intermediates (Pokorna and Zabranska, 2015). This chemo- trophic denitrification process inextricably couples sulfur oxidation with carbon and nitrogen metabolism, as illustrated in Figure 2. This group of bacteria can be found in reduced sulfur-rich environments, such as marine sediments and hydrothermal vents and plays a critical role in the global cycling of carbon, nitrogen, and sulfur (Barton et al., 2014).
Sulfur-metabolizing archaea are mainly present in high- temperature environments, such as sulfidic hot springs and deep-sea hydrothermal vents, including the Sulfolobales order (Table 1; Barton et al., 2014). Methanotrophic archaea that occur in sub-seafloor sediments commonly coexist with sulfate-reducing bacteria (SRB). Anaerobic methanotrophic archaea (ANME archaea) oxidize methane and provide reducing equivalents to SRB, while SRB reduce sulfate to sulfide and emit nitrogen gas. Dissimilatory sulfate reduction is coupled with methane oxidation and degradation of organic carbon compounds, as illustrated in Figure 2. Considering the high diversity of SRB in marine sediments and wetlands, they have an important role in the coupled biogeochemical cycling of carbon, nitrogen, and sulfur (Barton and Fauque, 2009; Wasmund et al., 2017; Bao et al., 2018).
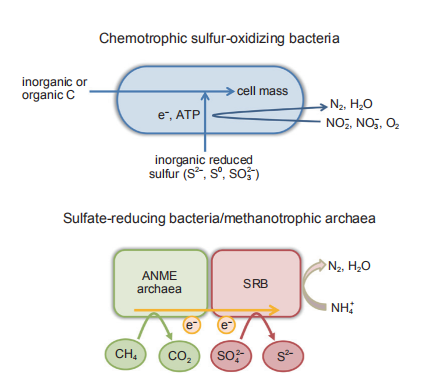
Figure 2 Coupled sulfur carbon and nitrogen metabolism in chemotrophic sulfur-oxidizing bacteria (SOB) and sulfate-reducing bacteria (SRB). Chemotrophic SOB can derive energy from the oxidation of a variety of reduced inorganic sulfur compounds and use oxidized forms of nitrogen as electron acceptors; thus, coupling sulfur oxidation with carbon and nitrogen transformation. SRB are able to coexist with methanotrophic archaea, coupling dissimilatory sulfate reduction with methane oxidation and degradation of organic carbon compounds.
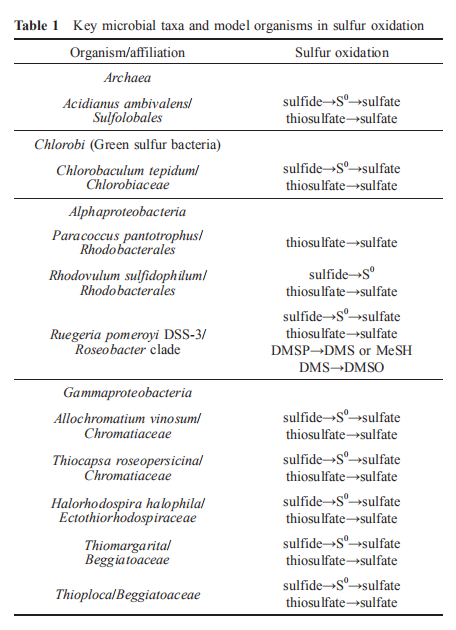
Despite the great phylogenetic and metabolic diversity of SOB, the most prominent members that carry out sulfur transformations belong to the classes of the Proteobacteria, including Alphaproteobacteria, Betaproteobacteria, and Gammaproteobacteria (Sievert et al., 2007; Walsh et al., 2009). Large non-phototrophic sulfur bacteria of the genera Beggiatoa, Thioploca, or Thiomargarita, possibly the most notable sulfur-oxidizing Gammaproteobacteria, store great quantities of elemental sulfur and nitrate in vacuoles, which can be used as electron acceptors and metabolic intermediates (Schulz and Jorgensen, 2001; Jorgensen, 2010). Beggiatoa species have been often detected in marine sediments of temperate coastal areas, e.g. Dangast/Wadden Sea (Mussmann et al., 2003) and Eckernforde Bay/Baltic Sea (Preisler et al., 2007). In anoxic sediments, marine Thioploca species convert nitrate to ammonia and fuel anaerobic oxidation, facilitating the recycling of bioavailable nitrogen (Winkel et al., 2014). Members of chemoautotrophic Epsi- lonproteobacteria are important sulfur-oxidizers in deep-sea hydrothermal vents (Sievert et al., 2007). In Epsilonproteo- bacteria, sulfur oxidation can be coupled to denitrification for energy conservation and reverse electron transport is proposed to produce ferredoxin for carbon fixation. The Epsilonproteobacteria group are also abundant in anoxic pelagic habitats and have key roles in global sulfur cyclingOctober (2018) Vol.61 No.10 and ecosystems (Grote et al., 2008; Lavik et al., 2009).
3.2 Marine heterotrophic sulfur-oxidizing bacteria
Most marine heterotrophic bacteria can also generate sulfide from sulfur-containing amino acids cysteine and methionine under aerobic conditions. Some bacteria harbor sulfide:qui- none oxidoreductase (SQR) or flavocytochrome c-sulfide dehydrogenase (FccAB) and oxidize self-produced sulfide, as shown in Table 2. Other bacteria without these enzymes produce sulfide and release the toxic gas H?S when it accumulates to high levels. The ubiquitous marine Roseobacter clade bacteria (RCB) in the Alphaproteobacteria class often carry the SQR, sulfite oxidase, and Sox systems that can completely oxidize sulfide, sulfite, and thiosulfate to sulfate (Lenk et al., 2012).
Marine Roseobacter clade bacteria (RCB) are widely distributed throughout the world's oceans. They are phylo- genetically coherent but physiologically diverse, comprising up to 25% of marine microbial communities, especially in coastal zones and polar regions (Brinkhoff et al., 2008). It has been suggested that RCB account for 3-11% of the total community of 16S rRNA gene pool in coastal sediment (Gonzalez and Moran, 1997). Given their worldwide distribution and versatile metabolic properties, RCB play a critical role in global biogeochemical processes, such as carbon and sulfur cycling (Moran et al., 2003). Pelagic RCB are involved in aerobic anoxygenic photosynthesis, oxidation of the greenhouse gas carbon monoxide, transformation of inorganic sulfur compounds, and production of the climate-relevant gas dimethyl sulfide (DMS) through the degradation of algal osmolyte DMSP (Wagner-Dobler and Biebl, 2006; Moran et al., 2007). Cultivation-based studies have revealed a variety of Roseobacter strains with the ability to oxidize inorganic sulfur compounds such as thiosulfate, sulfide, and sulfite. Isolated from the oxygen-sulfide interface of the Black Sea, the obligate heterotrophic bacteria Citreicella thiooxidans could oxidize sulfide and thiosulfate under aerobic conditions (Sorokin et al., 2005). Isolated from coastal seawater, the Silicibacterpo^ero^i is able to degrade DMSP, oxidize thiosulfate aerobically and use metabolic energy from inorganic sulfur oxidation (Gonzalez et al., 2003).
The marine algal osmoprotectant DMSP can be consumed by marine bacteria through two competing biochemical pathways, the demethylation pathway resulting in the release of methanethiol (MeSH) or the cleavage pathway producing the climate-active gas dimethyl sulfide (DMS) (Curson et al., 2011; Bullocket al., 2017). The volatile DMS is released into the atmosphere and then oxidized to acidic aerosol particles that aid in the formation of cloud condensation nuclei. DMS is the largest natural source of sulfur to the atmosphere and has a prominent role in the global sulfur cycle.
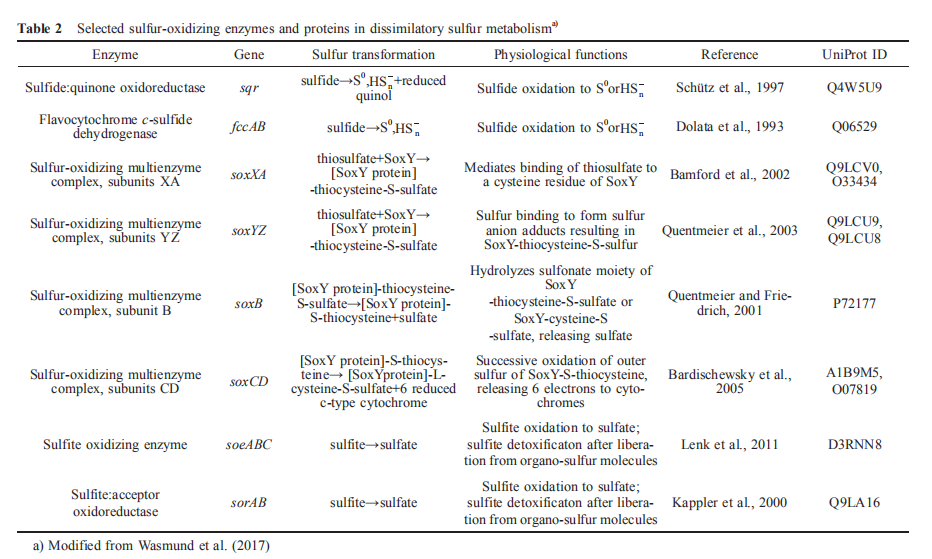
DMS can serve as a source of energy, carbon, and/or sulfur for heterotrophic, autotrophic, and phototrophic bacteria (Kappler and Schafer, 2014). Autotrophic Thiobacillus species (e.g., Thiobacillus thioparus Tk-m, Thiobacillus thio- parus T5) have been reported to degrade DMS under aerobic and denitrifying conditions. Anoxygenic phototrophic purple sulfur bacteria and phototrophic green sulfur bacteria can also oxidize DMS to DMSO, simultaneously the DMS oxidation process providing electrons for carbon fixation (Zeyer et al., 1987).
Some heterotrophic bacteria can also utilize DMS as a carbon or energy source (e.g., isolates of Delftia acidovorans and Sagittula stellata) (Zhang et al., 1991; Kappler and Schafer, 2014). A variety of aerobic methylotrophic bacteria are known to be able to grow on DMS as a sole carbon and energy source, including strains of Hyphomicrobium, Me- thylobacterium, Afipia, Arthrobacter, Methylophaga, and other bacteria (Kim et al., 2007; Schafer, 2007). Recently, Ruegeria pomeroyi DSS-3, the model marine heterotrophic bacteria in the Roseobacter clade, has been identified to oxidize DMS to DMSO using trimethylamine monooxygenase (Tmm). Tmm expression and DMS oxidation is mainly controlled at the post-transcriptional level. Tmm also occurs in another dominant bacterial community, the SAR11 clade, and occurs in approximately 20% of bacterial cells inhabiting the surface waters of the oceans. It has been suggested that DMS oxidation by Tmm-containing heterotrophic bacteria contributes to a notable proportion of the observed DMSO formation in marine surface waters (Lid- bury et al., 2016).
Another DMS-consuming enzyme is DMS monooxygenase, which converts DMS to MeSH and formaldehyde (Boden et al., 2011). DMS monooxygenase has been identified in Hyphomicrobium species, which are some of the earliest characterized DMS-degrading bacteria. DMS monooxygenase from Hyphomicrobium species is a flavindependent monooxygenase with two subunits. It has been reported that the enzyme activity can be decreased by chelation with ethylenediaminetetraacetic acid (EDTA) and bathocuproine (Kappler and Schafer, 2014).
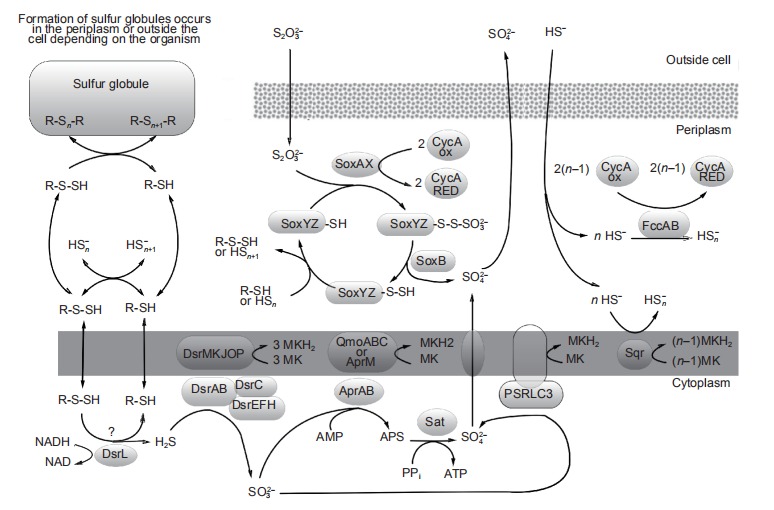
Figure 3 Overview of the central enzymes and pathways of sulfur oxidation in marine bacteria. Sulfide:quinone oxidoreductase (SQR) and flavocyto- chrome c (FccAB) oxidize sulfide to sulfane sulfur, and FccAB transfers electrons to cytochrome (CycA). The sulfur-oxidizing multienzyme system (SoxAX, SoxB, SoxCD and SoxYZ) is responsible for the oxidation of thiosulfate to sulfate. The reverse dissimilatory sulfite reductase (rDSR) pathway is indispensable to the oxidation of intermediately formed sulfur in SoxCD-deficient bacteria. The enzymes adenylylsulfate reductase (AprAB) and ATP sulfurylase (Sat) oxidize sulfite to sulfate. Modified from Frigaard and Dahl (2009).
4.The key enzymes and metabolic pathways involved in sulfur oxidation
Inorganic reduced sulfur compounds such as sulfide and sulfite can serve as electron donors to generate the proton motive force for adenosine triphosphate (ATP) production and facilitate aerobic respiration in heterotrophic bacteria (Marcia et al., 2010). The pathway of sulfur oxidation consists of three essential steps: the formation of elemental sulfur during oxidation of sulfide or thiosulfate; the oxidation of sulfide or elemental sulfur to sulfite; and the formation of sulfate as the final product. Figure 3 provides an overview of the proposed metabolic pathways and enzymes.
4.1 Oxidation of sulfide
Sulfide (H2S, HS-, and S2-) is the most reduced form of sulfur in the biogeochemical cycle, and is mainly produced by sulfate-reducing bacteria under anaerobic conditions, such as in the sulfate-reducing marine sediment or sulfate-methane transition zone (SMTZ) (Wasmund et al., 2017). In contrast, heterotrophic bacteria are able to utilize organic sulfur and release H2S under aerobic conditions. The sulfide produced may accumulate to high levels and harm bacterial growth. Many heterotrophic bacteria have evolved concerted actions of multiple enzymes to oxidize sulfide to sulfite and thiosulfate, and avoid the accumulation of toxic sulfide (Liu et al., 2014; Xin et al., 2016; Li et al., 2017).
In the initial step of sulfide oxidation, two main enzymes are involved, sulfide:quinone oxidoreductase and flavocy- tochrome c-sulfide dehydrogenase, as shown in Table 2. Sulfide:quinone oxidoreductase (SQR), an ancient membrane-bound flavoprotein, is a common enzyme for sulfide oxidation detoxification in a range of organisms, from mammals and invertebrates to chemotrophic and anaerobic photosynthetic bacteria (Reinartz et al., 1998). SQR, as a member of glutathione reductase family, is widely spread among autotrophic and heterotrophic bacteria, and has been extensively studied for decades. It has been investigated in detail in the cyanobacterium Oscillatoria limnetica and the proteobacterium Rhodobacter capsulatus over the last decade (Frigaard and Dahl, 2009). In both strains, the sqr genes have been cloned, sequenced and heterologously expressed in Escherichia coli. In Rhodobacter capsulatus, SQR binds to the membrane with its active site located in the periplasm (Griesbeck et al., 2002). According to the first X-ray structure of SQR which was determined to 2.6 A resolution, the enzyme has two redox active sites with a covalently bound flavin adenine dinucleotide (FAD) and an adjacent pair of cysteine residues (Brito et al., 2009). SQR catalyzes the oxidation of sulfide to sulfane sulfur, e.g., polysulfide, with ubiquinone as the electron acceptor, and passes the electrons to the electron transport system within the aerobic respiration process, which generates the proton motive force for ATP production (Schutz et al., 1999).
Flavocytochrome c-sulfide dehydrogenase (FccAB) can also oxidize sulfide to sulfane sulfur. The enzyme is mainly present in phototrophic bacteria and chemolithotrophic SOB, but appears less widespread than SQRs (Lu et al., 2017). In contrast to SQR, flavocytochrome c is usually soluble and periplasmic, consisting of a small FccA cytochrome c subunit about 20 kDa and a larger FccB flavoprotein subunit which can bind sulfide (Barton et al., 2014). FccA from Alc. vinosum is 21 kDa and binds two heme c, while in the green sulfur bacteria (GSB) FccA is only 10 kDa and binds a single heme (Verte et al., 2002). In a few strains of chemolitho- trophic bacteria, FccA and FccB are often referred as SoxE and SoxF because their genes occur in a conserved Sox gene cluster involved in thiosulfate oxidation (Gregersen et al., 2011). When soxE is not beside soxF, the gene coding SoxF homolog is also referred as soxJ. The purified monomeric flavoprotein SoxF exerts two functions in vitro', oxidizing sulfide and enhancing the thiosulfate oxidation activity of Sox system (Ogawa et al., 2010). In vitro, flavocytochrome c can catalyze the oxidization of sulfide to zero valence sulfur, and pass the electrons to a variety of small c-type cytochromes e.g., Alc. vinosum cytochrome c550, while SQRs pass electrons from sulfide oxidation into the electron transport chain via ubiquinone. The physiological role of flavocytochrome c remains debatable. If indeed the flavo- cytochrome c oxidizes sulfide in vivo, both green and purple sulfur bacteria apparently have alternative sulfide-oxidizing enzymes such as SQR that may be more efficient in conserving energy. However, it is also possible that flavocyto- chrome c, which has high affinity for sulfide oxidation and can donate electrons to the photosynthetic reaction center finally, is of advantage for the cells especially at very low sulfide concentrations.
4.2 Oxidation of thiosulfate to sulfate
Thiosulfate is a rather stable and common sulfur source for many SOB. The sulfur-oxidizing multienzyme system (Sox system) is a periplasmic multienzyme complex responsible for thiosulfate oxidation to sulfate as shown in Table 2 and Figure 3. It was first described and characterized in the al- phaproteobacterium Paracoccus (Pc.) versutus and Pc. pantotrophus which are facultative chemolithoautotrophs and utilize thiosulfate as their sole energy source (Friedrich et al., 2001). The Sox multienzyme system is essential for the oxidation of thiosulfate to sulfate and has been observed in all the thiosulfate-oxidizing strains distributed over a wide range of phylogenetic lineages (Alpha-, Beta-, Gamma-, Epsilonproteobacteria) (Friedrich et al., 2005). The sox gene cluster comprises 15 genes (soxRSVWXYZABCDEFGH), with the core gene cluster soxXYZABCD encoding four periplasmic proteins SoxAX, SoxYZ, SoxB, and Sox(CD)2. The SoxAX cytochromes are heme-thiolate proteins, which initiate the reaction cycle of Sox complex by catalyzing the attachment of sulfur substrates, such as thiosulfate to a conserved cysteine (Kappler and Maher, 2013). The SoxYZ complex does not contain any cofactor and covalently binds sulfur compounds of various oxidation states to a conserved cysteine residue (Quentmeier and Friedrich, 2001). The monomeric SoxB, containing a dinuclear manganese cluster (Epel et al., 2005), has been shown to interact with the SoxYZ complex and is believed to function as a sulfate thiohydrolase (Quentmeier et al., 2003). The Sox(CD)2 complex, composed of the molybdoprotein SoxC and the diheme c-type cytochrome SoxD, is dispensable in GSB and some marine Gamma sulfur oxidizing bacteria (Quentmeier et al., 2000). Those bacteria deficient of SoxCD oxidize the intermediary sulfur via reverse dissimilatory sulfite reductase (rDSR). Enzymes of the rDSR pathway play the main role in the oxidation of the intermediary sulfur deposits and compensate for the absence of SoxCD in that group of bacteria (Frigaard and Dahl, 2009).
5. Concluding remarks
Sulfur is a major ecological and evolutionary driver of microbial life in the ocean. Inorganic sulfur compounds serve as electron acceptors and donors for microorganisms to harness energy. Sulfur cycling is coupled to the cycling of carbon and nitrogen through microbe-mediated redox processes. Recent findings have revealed that microorganisms have evolved remarkably diverse genetic and metabolic features to fulfil an array of ecological niches in various marine habitats. Meta- genomic approaches have contributed new discoveries on uncultured bacteria. Integrating molecular biological approaches and other 'omics' technologies will provide novel opportunities and insights in revealing the metabolic capabilities and potential of sulfur-transforming bacteria. Me- socosm has been widely applied by imitating natural conditions, which greatly improves our understanding on the processes and mechanisms in the ocean at the ecosystem level. Recently, a fully controlled experimental systems is proposed, named Marine Environmental Chambers System (MECS), which can provide a much more advanced platform to study the complicated processes occurring in the ocean, especially for carbon cycling, sulfur cycling, and the interactions between each cycle in specific oceanic scenarios (Jiao et al., 2010, 2014; Legendre et al., 2018).
Currently, seasonal or persistent hypoxia extends throughout many coastal areas and causes accumulations of toxic hydrogen sulfide that arises from the sediment, which could have serious consequences for coastal ecosystems and economies. The complete sulfur oxidation by heterotrophic bacteria can convert hydrogen sulfide to sulfate and protect marine organisms from sulfide toxicity. The application and exploitation of biological sulfide detoxification technology mediated by marine microorganisms has great significance for the maintenance and protection of coastal ecosystems. Although our understanding of sulfur cycling processes and the variety of microbial communities has improved considerably, many important questions remain to be answered regarding the metabolic mechanisms of sulfur-transforming bacteria and the factors that control the turnover of sulfur compounds in biogeochemical cycles. The characterization of unknown sulfur-converting enzymes and biochemical pathways will enable us to interpret the essential functions of sulfur-transforming bacteria in the marine sulfur and carbon cycles.
Acknowledgements This work was supported by the National Key Research and Development Program of China (Grant No. 2016YFA0601103), the National Natural Science Foundation of China (Grant No. 41606134) and the Fundamental Research Funds of Shandong University as well.
References
Bamford V A, Bruno S, Rasmussen T, Appia-Ayme C, Cheesman M R, Berks B C, Hemmings A M. 2002. Structural basis for the oxidation of thiosulfate by a sulfur cycle enzyme. Embo J, 21: 5599-5610
Bao P,LiGX,SunGX,XuYY, Meharg A A, Zhu Y G. 2018. The role of sulfate-reducing prokaryotes in the coupling of element biogeochemical cycling. Sci Total Environ, 613-614: 398-408
Bardischewsky F, Quentmeier A, Rother D, Hellwig P, Kostka S, Friedrich C G. 2005. Sulfur dehydrogenase of Paracoccus pantotrophus: The heme-2 domain of the molybdoprotein cytochrome c complex is dispensable for catalytic activity. Biochemistry, 44: 7024-7034
Barton L L, Fardeau M L, Fauque G D. 2014. Hydrogen sulfide: A toxic gas produced by dissimilatory sulfate and sulfur reduction and consumed by microbial oxidation. Met Ions Life Sci, 14: 237-277
Barton L L, Fauque G D. 2009. Biochemistry, physiology and biotechnology of sulfate-reducing bacteria. Adv Appl Microbiol, 68: 41-98 Boden R, Borodina E, Wood A P, Kelly D P, Murrell J C, Scha fer H. 2011.
Purification and characterization of dimethylsulfide monooxygenase from hyphomicrobium sulfonivorans. J Bacteriol, 193: 1250-1258 Bowles M W, Mogollon J M, Kasten S, Zabel M, Hinrichs K U. 2014.
Global rates of marine sulfate reduction and implications for sub-seafloor metabolic activities. Science, 344: 889-891
Brinkhoff T, Giebel H A, Simon M. 2008. Diversity, ecology, and genomics of the Roseobacter clade: A short overview. Arch Microbiol, 189: 531539
Brito J A, Sousa F L, Stelter M, Bandeiras T M, Vonrhein C, Teixeira M, Pereira M M, Archer M. 2009. Structural and functional insights into sulfide: Quinone oxidoreductase. Biochemistry, 48: 5613-5622
Bruser T, Lens P N L, Truper H G. 2000. The biological sulfur cycle. In: Lens P N L, Pol L, eds. Environmental Technologies to Treat Sulfur Pollution. London: IWA Publishing
Bullock H A, Luo H, Whitman W B. 2017. Evolution of dimethylsulfo- niopropionate metabolism in marine phytoplankton and bacteria. Front Microbiol, 8: e0127288
Curson A R J, Todd J D, Sullivan M J, Johnston A W B. 2011. Catabolism of dimethylsulphoniopropionate: Microorganisms, enzymes and genes. Nat Rev Microbiol, 9: 849-859
Diaz R J, Rosenberg R. 2008. Spreading dead zones and consequences for marine ecosystems. Science, 321: 926-929
Dolata M M, Van Beeumen J J, Ambler R P, Meyer T E, Cusanovich M A. 1993. Nucleotide sequence of the heme subunit of flavocytochrome c from the purple phototrophic bacterium, Chromatium vinosum. A 2.6- kilobase pair DNA fragment contains two multiheme cytochromes, a flavoprotein, and a homolog of human ankyrin. J Biol Chem, 268: 14426-14431
Durham B P, Sharma S, Luo H, Smith C B, Amin S A, Bender S J, Dearth S P, Van Mooy BAS, Campagna S R, Kujawinski E B, Armbrust E V, Moran M A. 2015. Cryptic carbon and sulfur cycling between surface ocean plankton. Proc Natl Acad Sci USA, 112: 453-457
Epel B, Schafer K O, Quentmeier A, Friedrich C, Lubitz W. 2005. Multifrequency EPR analysis of the dimanganese cluster of the putative sulfate thiohydrolase SoxB of Paracoccus pantotrophus. J Biol Inorg Chem, 10: 636-642
Friedrich C G, Bardischewsky F, Rother D, Quentmeier A, Fischer J. 2005. Prokaryotic sulfur oxidation. Curr Opin Microbiol, 8: 253-259
Friedrich C G, Rother D, Bardischewsky F, Quentmeier A, Fischer J. 2001. Oxidation of reduced inorganic sulfur compounds by bacteria: Emergence of a common mechanism? Appl Environ Microbiol, 67: 2873- 2882
Frigaard N U, Dahl C. 2009. Sulfur metabolism in phototrophic sulfur bacteria. Adv Microb Physiol, 54: 103-200
Gonzalez J M, Covert J S, Whitman W B, Henriksen J R, Mayer F, Scharf
B,Schmitt R, Buchan A, Fuhrman J A, Kiene R P, Moran M A. 2003. Silicibacter pomeroyi sp. nov. and Roseovarius nubinhibens sp. nov., dimethylsulfoniopropionate-demethylating bacteria from marine environments. Int J Syst Evol Microbiol, 53: 1261-1269
Gonzalez J M, Kiene R P, Moran M A. 1999. Transformation of sulfur compounds by an abundant lineage of marine bacteria in the a-Subclass of the class proteobacteria. Appl Environ Microbiol, 65: 3810-3819
Gonzalez J M, Moran M A. 1997. Numerical dominance of a group of marine bacteria in the alpha-subclass of the class Proteobacteria in coastal seawater. Appl Environ Microbiol, 63: 4237-4242
Gregersen L H, Bryant D A, Frigaard N U. 2011. Mechanisms and evolution of oxidative sulfur metabolism in green sulfur bacteria. Front Microbiol, 2: 116
Griesbeck C, Schutz M, Schodl T, Bathe S, Nausch L, Mederer N, Viel- reicher M, Hauska G. 2002. Mechanism of sulfide-quinone reductase investigated using site-directed mutagenesis and sulfur analysis. Biochemistry, 41: 11552-11565
Grote J, Jost G, Labrenz M, Herndl G J, Jurgens K. 2008. Epsilonproteo- bacteria represent the major portion of chemoautotrophic bacteria in sulfidic waters of pelagic redoxclines of the Baltic and Black Seas. Appl Environ Microbiol, 74: 7546-7551
Howard E C, Sun S, Biers E J, Moran M A. 2008. Abundant and diverse bacteria involved in DMSP degradation in marine surface waters. Environ Microbiol, 10: 2397-2410
Jiao N, Herndl G J, Hansell D A, Benner R, Kattner G, Wilhelm S W, Kirchman D L, Weinbauer M G, Luo T, Chen F, Azam F. 2010. Microbial production of recalcitrant dissolved organic matter: Long-term carbon storage in the global ocean. Nat Rev Microbiol, 8: 593-599
Jiao N, Robinson C, Azam F, Thomas H, Baltar F, Dang H, Hardman- Mountford N J, Johnson M, Kirchman D L, Koch B P, Legendre L, Li
C,Liu J, Luo T, Luo Y W, Mitra A, Romanou A, Tang K, Wang X, Zhang C, Zhang R. 2014. Mechanisms of microbial carbon sequestration in the ocean一Future research directions. Biogeosciences, 11: 5285-5306
Jorgensen B B. 1982. Mineralization of organic matter in the sea bed一The role of sulphate reduction. Nature, 296: 643-645
Jorgensen B B. 2010. Big sulfur bacteria. Isme J, 4: 1083-1084
Jorgensen B B, Nelson D C. 2004. Sulfide oxidation in marine sediments: Geochemistry meets microbiology. Geol Soc Am Spec Pap, 379: 63-81
Kai F, Liesack W, Bo T. 1998. Elemental sulfur and thiosulfate disproportionation by Desulfocapsa sufoexigens sp. nov. a new anaerobic bacterium isolated from marine surface sediment. Appl Environ Microbiol, 64: 119-125
Kappler U, Bennett B, Rethmeier J, Schwarz G, Deutzmann R, McEwan A G, Dahl C. 2000. Sulfite:Cytochromec oxidoreductase fromThiobacillus novellus. J Biol Chem, 275: 13202-13212
Kappler U, Maher M J. 2013. The bacterial SoxAX cytochromes. Cell Mol Life Sci, 70: 977-992
Kappler U, Schafer H. 2014. Transformations of dimethyl sulfide. In: The Metal-Driven Biogeochemistry of Gaseous Compounds in the Environment. Springer Netherlands. 279-313
Kim H G, Doronina N V, Trotsenko Y A, Kim S W. 2007. Methylophaga
aminisulfidivorans sp. nov., a restricted facultatively methylotrophic marine bacterium. Int J Syst Evol Microbiol, 57: 2096-2101
Lavik G, Stuhrmann T, Bruchert V, Van der Plas A, Mohrholz V, Lam P, Mussmann M, Fuchs B M, Amann R, Lass U, Kuypers M MM. 2009. Detoxification of sulphidic African shelf waters by blooming chemo- lithotrophs. Nature, 457: 581-584
Legendre L, Rivkin R B, Jiao N. 2018. Advanced experimental approaches to marine water-column biogeochemical processes. Ices J Mar Sci, 75: 30-42
Leloup J, Fossing H, Kohls K, Holmkvist L, Borowski C, Jorgensen B B. 2009. Sulfate-reducing bacteria in marine sediment (Aarhus Bay, Denmark): Abundance and diversity related to geochemical zonation. Environ Microbiol, 11: 1278-1291
Lenk S. 2011. Molecular ecology of key organisms in sulfur and carbon cycling in marine sediments. Dissertation for Doctoral Degree. Bremen: Max Planck Institute for Marine Microbiology
Lenk S, Arnds J, Zerjatke K, Musat N, Amann R, Mussmann M. 2011. Novel groups of Gammaproteobacteria catalyse sulfur oxidation and carbon fixation in a coastal, intertidal sediment. Environ Microbiol, 13: 758-774
Lenk S, Moraru C, Hahnke S, Arnds J, Richter M, Kube M, Reinhardt R, Brinkhoff T, Harder J, Amann R, MuBmann M. 2012. Roseobacter clade bacteria are abundant in coastal sediments and encode a novel combination of sulfur oxidation genes. Isme J, 6: 2178-2187
Li C, Cheng M, Algeo T J, Xie S C. 2015. A theoretical prediction of chemical zonation in early oceans (>520 Ma). Sci China Earth Sci, 58: 1901-1909
Li H, Li J, Lu C, Xia Y, Xin Y, Liu H, Xun L, Liu H. 2017. FisR activates c54-dependent transcription of sulfide-oxidizing genes in Cupriavidus pinatubonensis JMP134. Mol Microbiol, 105: 373-384
Lidbury I, Krober E, Zhang Z, Zhu Y, Murrell J C, Chen Y, Schafer H. 2016. A mechanism for bacterial transformation of dimethyl sulfide to dimethyl sulfoxide: A missing link in the marine organic sulfur cycle. Environ Microbiol, 18: 2754-2766
Lin X, Wakeham S G, Putnam I F, Astor Y M, Scranton M I, Chistoserdov A Y, Taylor G T. 2006. Comparison of vertical distributions of prokaryotic assemblages in the anoxic Cariaco Basin and Black Sea by use of fluorescence in situ hybridization. Appl Environ Microbiol, 72: 2679-2690
Liu H, Xin Y, Xun L. 2014. Distribution, diversity, and activities of sulfur dioxygenases in heterotrophic bacteria. Appl Environ Microbiol, 80: 1799-1806
Luther G W, Findlay A J, MacDonald D J, Owings S M, Hanson T E, Beinart R A, Girguis P R. 2011. Thermodynamics and kinetics of sulfide oxidation by oxygen: A look at inorganically controlled reactions and biologically mediated processes in the environment. Front Microbio, 2: 62
Lu C, Xia Y, Liu D, Zhao R, Gao R, Liu H, Xun L. 2017. Cupriavidus necator H16 uses flavocytochrome c-sulfide dehydrogenase to oxidize self-produced and added sulfide. Appl Environ Microbiol, 83: e01610- 17
Mangold S, Valdes J, Holmes D S, Dopson M. 2011. Sulfur metabolism in the extreme acidophile Acidithiobacillus caldus. Front Microbiol, 2: 17
Marcia M, Ermler U, Peng G, Michel H. 2010. A new structure-based classification of sulfide: quinone oxidoreductases. Proteins, 78: 10731083
Moran M A, Belas R, Schell M A, Gonzalez J M, Sun F, Sun S, Binder B J, Edmonds J, Ye W, Orcutt B, Howard E C, Meile C, Palefsky W, Goesmann A, Ren Q, Paulsen I, Ulrich L E, Thompson L S, Saunders E, Buchan A. 2007. Ecological genomics of marine Roseobacters. Appl Environ Microbiol, 73: 4559-4569
Moran M A, Gonzalez J M, Kiene R P. 2003. Linking a bacterial taxon to sulfur cycling in the sea: Studies of the marine Roseobacter group. Geomicrobiol J, 20: 375-388
Mussmann M, Schulz H N, Strotmann B, Kjaer T, Nielsen L P, Rossello- Mora R A, Amann R I, Jorgensen B B. 2003. Phylogeny and distribution of nitrate-storing Beggiatoa spp. in coastal marine sediments.
Environ Microbiol, 5: 523-533
Ogawa T, Furusawa T, Shiga M, Seo D, Sakurai H, Inoue K. 2010. Biochemical studies of a soxF-encoded monomeric flavoprotein purified from the green sulfur bacterium Chlorobaculum tepidum that Stimulates in Vitro thiosulfate oxidation. Biosci Biotech Biochem, 74: 771-780
Pokorna D, Zabranska J. 2015. Sulfur-oxidizing bacteria in environmental technology. Biotech Adv, 33: 1246-1259
Preisler A, de Beer D, Lichtschlag A, Lavik G, Boetius A, Jorgensen B B. 2007. Biological and chemical sulfide oxidation in a Beggiatoa inhabited marine sediment. Isme J, 1: 341-353
Quentmeier A, Friedrich C G. 2001. The cysteine residue of the SoxY protein as the active site of protein-bound sulfur oxidation of Paracoccus pantotrophus GB17. Febs Lett, 503: 168-172
Quentmeier A, Hellwig P, Bardischewsky F, Grelle G, Kraft R, Friedrich C G. 2003. Sulfur oxidation in Paracoccus pantotrophus: Interaction of the sulfur-binding protein SoxYZ with the dimanganese SoxB protein. Biochem Biophys Res Commun, 312: 1011-1018
Quentmeier A, Kraft R, Kostka S, Klockenkamper R, Friedrich C G. 2000. Characterization of a new type of sulfite dehydrogenase from Paracoccus pantotrophus GB17. Arch Microbiol, 173: 117-125
Reinartz M, Tschape J, Bruser T, Truper H G, Dahl C. 1998. Sulfide oxidation in the phototrophic sulfur bacterium Chromatium vinosum. Archives Microbiol, 170: 59-68
Schafer H. 2007. Isolation of Methylophaga spp. from marine dimethylsulfide-degrading enrichment cultures and identification of polypeptides induced during growth on dimethylsulfide. Appl Environ Microbiol, 73: 2580-2591
Schulz H N, Jorgensen B B. 2001. Big bacteria. Annu Rev Microbiol, 55: 105-137
Schutz M, Maldener I, Griesbeck C, Hauska G. 1999. Sulfide-quinone reductase from Rhodobacter capsulatus: Requirement for growth, periplasmic localization, and extension of gene sequence analysis. J Bacteriol, 181: 6516-6523
Schutz M, Shahak Y, Padan E, Hauska G. 1997. Sulfide-quinone reductase from Rhodobacter capsulatus. Purification, cloning, and expression. J Biol Chem, 272: 9890-9894
Selman M, Greenhalgh S, Diaz R, Sugg, Z. 2008. Eutrophication and hypoxia in coastal areas: A global assessment of the state of knowledge. J Am Geriatr Soc, 51: 1305-1317
Sievert S, Kiene R, Schulz-Vogt H. 2007. The sulfur cycle. Oceanography, 20: 117-123
Sorokin D Y, Tourova T P, Muyzer G. 2005. Citreicella thiooxidans gen. nov., sp. nov., a novel lithoheterotrophic sulfur-oxidizing bacterium from the Black Sea. Syst Appl Microbiol, 28: 679-687
Thamdrup B, Finster K, Hansen J W, Bak F. 1993. Bacterial disproportionation of elemental sulfur coupled to chemical reduction of iron or manganese. Appl Environ Microbiol, 59: 101-108
Verte F, Kostanjevecki V, De Smet L, Meyer T E, Cusanovich M A, Van Beeumen J J. 2002. Identification of a thiosulfate utilization gene cluster from the green phototrophic bacterium Chlorobium limicola. Biochemistry, 41: 2932-2945
Wagner-Dobler I, Biebl H. 2006. Environmental Biology of the marine Roseobacter lineage. Annu Rev Microbiol, 60: 255-280
Walsh D A, Zaikova E, Howes C G, Song Y C, Wright J J, Tringe S G, Tortell P D, Hallam S J. 2009. Metagenome of a versatile chemo- lithoautotroph from expanding oceanic dead zones. Science, 326: 578582
Wasmund K, MuBmann M, Loy A. 2017. The life sulfuric: Microbial ecology of sulfur cycling in marine sediments. Environ Microbol Rep, 9: 323-344
Winkel M, de Beer D, Lavik G, Peplies J, MuBmann M. 2014. Close association of active nitrifiers with Beggiatoa mats covering deep-sea hydrothermal sediments. Environ Microbiol, 16: 1612-1626
Xia Y, Lu C, Hou N, Xin Y, Liu J, Liu H, Xun L. 2017. Sulfide production and oxidation by heterotrophic bacteria under aerobic conditions. Isme J, 11: 2754-2766
Xie S C, Chen J F, Wang F P, Xun L Y, Tang K, Zhai W D, Liu J H, Ma W
T. 2017. Mechanisms of carbon storage and the coupled carbon, nitrogen and sulfur cycles in regional seas in response to global change. Sci China Earth Sci, 60: 1010-1014
Xin Y, Liu H, Cui F, Liu H, Xun L. 2016. Recombinant Escherichia coli with sulfide:quinone oxidoreductase and persulfide dioxygenase rapidly oxidises sulfide to sulfite and thiosulfate via a new pathway. Environ Microbiol, 18: 5123-5136
Zaikova E, Walsh D A, Stilwell C P, Mohn W W, Tortell P D, Hallam S J. 2009. Microbial community dynamics in a seasonally anoxic fjord: Saanich Inlet, British Columbia. Environ Microbiol, 12: 172-191
Zeyer J, Eicher P, Wakeham S G, Schwarzenbach R P. 1987. Oxidation of dimethyl sulfide to dimethyl sulfoxide by phototrophic purple bacteria. Appl Environ Microbiol, 53: 2026-2032
Zhang L, Kuniyoshi I, Hirai M, Shoda M. 1991. Oxidation of dimethyl sulfide by Pseudomonas acidovorans DMR-11 isolated from peat biofilter. Biotechnol Lett, 13: 223-228
(Responsible editor: Xiaoxue WANG)